You are here: Home > Section on Nervous System Development and Plasticity
Nervous System Development and Plasticity
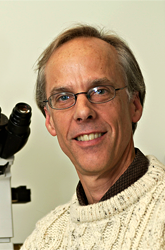
- R. Douglas Fields, PhD, Head, Section on Nervous System Development and Plasticity
- Philip Lee, PhD, Staff Scientist
- Konstantina Psachoulia, PhD, Visiting Fellow
- Michelle Klippel, BS, Technician Biologist
- Olena Bukalo, PhD, Special Volunteer
Healthy brain and cognitive development in children is central to the mission of NICHD. Unlike the brains of most animals, the human brain continues to develop postnatally, through adolescence and into early adulthood. The prolonged postnatal period of brain development allows environmental experiences to influence brain structure and function, rather than having brain function specified entirely by genes. Activity-dependent plasticity also compensates for developmental defects and brain injury. Our research is concerned with understanding the molecular and cellular mechanisms by which functional activity in the brain regulates development of the nervous system during late stages of fetal development and early postnatal life. We are especially interested in exploring new mechanisms of activity-dependent nervous system plasticity that are particularly relevant to the period of childhood. The work has three main areas of emphasis.
Myelination and neuron-glia interactions:
Traditionally, the field of activity-dependent nervous system development has focused on synapses, and we continue to explore synaptic plasticity; however, our research is also advancing understanding of how non-neuronal brain cells (glia) sense neural impulse activity and how activity-dependent regulation of glia contributes to development, plasticity, and the cellular mechanisms of learning. A major emphasis of our current research is to understand how myelin (white matter in the brain) is regulated by functional activity. By changing conduction velocity, activity-dependent myelination may be a non-synaptic form of plasticity regulating nervous system function by optimizing the speed and synchrony of information transmission through neural networks. Our studies identified several cellular and molecular mechanisms for activity-dependent myelination, and the findings have important implications for normal brain development, learning and cognition, and psychiatric disorders. Our research showing that myelination of axons by glia (oligodendrocytes and Schwann cells) is regulated by impulse activity provides evidence for a new form of nervous system plasticity and learning that would be particularly important in child development, given that myelination proceeds throughout childhood and adolescence. The mechanisms we identified suggest that environmental experience may alter myelin formation in an activity-dependent manner, thereby improving function based on experience.
Cellular mechanisms of learning:
Learning is perhaps the most important function of childhood. Our research is delineating the molecular mechanisms that convert short-term memory into long-term memory. While we continue our long-standing research on synaptic plasticity, our laboratory is actively exploring new mechanisms of nervous system plasticity during learning that extend beyond the neuron doctrine, such as neurons firing antidromically and the release of neurotransmitters along axons. We are investigating how gene expression necessary for long-term memory is controlled and how intrinsic activity in the brain (oscillations and neuronal firing) forms memories. Our recent research showed that neurons in the hippocampus fire backwards (antidromically) during sharp-wave ripple complexes, which are most frequent during slow-wave sleep, and that the firing reduces the strength of all synapses on that neuron (AP-LTD).
Gene regulation by neuronal firing:
Information in the nervous system is encoded in the temporal pattern of action potential firing. If functional experiences produce lasting effects on brain development and plasticity, specific genes must be regulated by specific patterns of impulse firing. We verified the hypothesis and are determining how different patterns of neural impulses regulate specific genes controlling development and plasticity of the nervous system and how impulse activity affects neurons and glia.
Regulation of myelination by neural impulse activity
Myelin, the multilayered membrane of insulation wrapped around nerve fibers (axons) by glial cells (oligodendrocytes), is essential for nervous system function, increasing conduction velocity at least 50-fold. Myelination is an essential part of brain development. The processes controlling myelination of appropriate axons are not well understood. Myelination begins in late fetal life and continues throughout childhood and adolescence, but myelination of some brain regions is not complete until an individual's early twenties. Our research shows that neurotransmitters that are released along axons firing action potentials activate receptors on myelinating glia (Schwann cells in the peripheral nervous system and oligodendrocytes in the central nervous system) as well as astrocytes and other cells, which in turn release growth factors or cytokines that regulate development of myelinating glia.
In addition to establishing the effects of impulse activity on proliferation and development of myelinating glia, we determined that release of the neurotransmitter glutamate from vesicles along axons promotes the initial events in myelin induction, including stimulating the formation of cholesterol-rich signaling domains between oligodendrocytes and axons and increasing the local synthesis of myelin basic protein, the major protein in the myelin sheath, through Fyn kinase–dependent signaling. Axon-oligodendrocyte signaling would thus promote myelination of electrically active axons to regulate neural development and to function according to environmental experience. The findings are also relevant to such demyelinating disorders as multiple sclerosis and to remyelination after axon injury.
We also found that other signaling molecules released from axons, notably ATP, stimulate differentiation of oligodendrocytes and increase myelination. In collaboration with colleagues in Italy, we found that GPR-17, a new membrane receptor on oligodendrocyte progenitor cells, regulates oligodendrocyte differentiation.
The release of neuronal messengers outside synapses has broad biological implications, particularly with regard to communication between axons and glia. We identified a mechanism for nonsynaptic, nonvesicular release of ATP from axons through volume-activated anion channels (VAACs), which are activated by microscopic axon swelling during action potential firing. The studies combine imaging of single photons to measure ATP release in a luciferin/luciferase assay with imaging of intrinsic optical signals, intracellular calcium, time-lapse video, and confocal microscopy. Microscopic axon swelling accompanying electrical depolarization of axons activates the VAACs to release ATP. Such nonvesicular, nonsynaptic communication may mediate various activity-dependent interactions between axons and nervous system cells under normal conditions, during development, and in disease.
Although the significance of myelin has been traditionally viewed in terms of conduction failure and spike time arrival in determining synaptic function and plasticity, we are exploring the importance of myelination in affecting the frequency, phase, and amplitude coupling of oscillations in the brain, as well as the propagation of brain waves. Abnormalities in brain waves and synchrony are associated with many psychiatric and developmental conditions, including, among others, schizophrenia, epilepsy, dyslexia, and autism.
Synaptic plasticity
To maintain the general level of neural impulse activity within normal limits, homeostatic mechanisms are required to control the formation and maintenance of synaptic connections during development and learning. How genes controlling these processes are coordinately regulated during homeostatic synaptic plasticity is unknown. Micro RNAs (miRNAs) exert regulatory control over mRNA stability and protein translation and may contribute to local activity-dependent post-transcriptional control of synapse-associated mRNAs. Using a bioinformatics screen to search for sequence motifs enriched in the 3′ UTR of mRNAs that are rapidly destabilized after increasing impulse activity in hippocampal neurons, we identified a developmentally and activity-regulated miRNA (miR-485) and showed that it controls dendritic spine number and synapse formation in an activity-dependent, homeostatic manner. Many plasticity-associated genes contain predicted miR-485–binding sites, including ones for the presynaptic protein SV2A. We found that miR-485 reduces SV2A abundance and negatively regulates dendritic spine density, clustering of the post-synaptic density protein (PSD-95), and surface expression of the glutamate receptor GluR2. Overexpression of miR-485 reduces spontaneous synaptic responses and transmitter release, as measured by miniature excitatory postsynaptic current analysis and FM 1-43 staining. The findings demonstrate that miRNAs participate in homeostatic synaptic plasticity, with possible implications for neurological disorders such as Huntington's and Alzheimer's disease, where miR-485 has been found to be dysregulated.
Hippocampal synaptic plasticity
It is widely appreciated that there are two types of memory: short-term and long-term. It has been known for decades that gene expression is necessary to convert short-term into long-term memory, but it is not known how signals reach the nucleus to initiate this process or which genes make memories permanent. Long-term potentiation (LTP) and long-term depression (LTD) are two widely studied forms of synaptic plasticity that can be recorded electrophysiologically in the hippocampus and are believed to represent a cellular basis for memory. We use cDNA microarrays to investigate the signaling pathways, genes, and proteins involved in LTP and LTD. The work is contributing to a better understanding of how regulatory networks are controlled by the appropriate patterns of impulses, leading to different forms of synaptic plasticity, and identifying new molecular mechanisms regulating synaptic strength.
In contrast to sensory-evoked stimulation, intrinsic activity in the brain often operates in non-traditional modes. We demonstrated how non-traditional modes of neuronal firing in the hippocampus during high-frequency oscillations (sharp-wave ripple complexes) affect synaptic plasticity in the process of memory consolidation during slow-wave sleep. During slow-wave sleep and periods of quiet wakefulness, CA1 neurons in the hippocampus fire backwards (antidromically) during brief high-frequency oscillations called sharp-wave ripple complexes. The action potential is initiated in the distal axon and propagates back into the cell body and dendrites. Our studies show that this antidromic firing reduces the strength of all synaptic inputs to the neuron (action potential-induced long-term depression [AP-LTD]) and that the synapses then become sensitized to being strengthened by subsequent sensory input. The process of globally reducing synaptic strength participates in the formation of transiently stable functional assemblies of neurons. The process is necessary for incorporating new information together with existing memories to form a schema, or coherent memory, combining multiple sensations and temporal sequence into a cognitive framework. AP-LTD, this new form of synaptic plasticity, may contribute to memory consolidation by sharpening specificity of subsequent synaptic input and promoting incorporation of novel information.
Regulation of gene expression by action-potential firing patterns
To determine how gene expression in neurons and glia is regulated by impulse firing, we stimulate nerve cells to fire impulses in differing patterns by delivering electrical stimulation through platinum electrodes in specially designed cell culture dishes. After stimulation, we measured mRNA and protein expression by gene arrays, quantitative RT-PCR (reverse transcriptase–polymerase chain reaction), Western blot, and immunocytochemistry. The results confirm our hypothesis that precise patterns of impulse activity can increase or decrease expression of specific genes (in neurons and glia). The experiments are revealing signaling and gene-regulatory networks that respond selectively to appropriate temporal patterns of action potential firing. Temporal aspects of intracellular calcium signaling are particularly important in regulating gene expression according to neural impulse firing patterns in normal and pathological conditions. Our findings thus provide a deeper understanding of how nervous system development and plasticity are regulated by information coded in the temporal pattern of impulse firing in the brain. The findings are also relevant to chronic pain as well as to the regulation of nervous system development and myelination by functional activity.
Additional Funding
- NSF grant SMA-1258562, awarded Sept. 19, 2012, to Dr. Fields as Co-PI with Dr. Beth Stevens, Harvard Medical School
- DOD Grant: 2012, Gulf War Illness Research Program Consortium Award, CDMRP Number: GW120037, Project Duration: 48 months
Publications
- Fields RD. Map the other brain. Nature 2013;501:25-27.
- Bukalo O, Campanac E, Hoffman DA, Fields RD. Synaptic plasticity by antidromic firing during hippocampal network oscillations. Proc Natl Acad Sci USA 2013;110:5175-5180.
- Zatorre RJ, Fields RD, Johansen-Berg H. Plasticity in gray and white: neuroimaging changes in brain structure during learing. Nat Neurosci 2012;15:528-536.
- Pajevic S, Basser P, Fields RD. Role of myelin plasticity in oscillations and synchrony of neuronal activity. Neuroscience 2013;in press.
- Fields RD, Araque A, Johansen-berg H, Lim S-S, Lynch G, Nave K-A, Nedergaard M, PerezR, Sejnowski T, Wake H. Glial biology in learning and cognition. Neuroscientist 2013;Epub ahead of print.
Collaborators
- Maria Pia Abbracchio, PhD, University of Milan
- Alfonso Araque, PhD, Instituto Cajal, Madrid, Spain
- Peter J. Basser, PhD, Program on Pediatric Imaging and Tissue Sciences, NICHD, Bethesda, MD
- Emilie A. Campanac, PhD, Program in Developmental Neuroscience, NICHD, Bethesda, MD
- Patrizia Casaccia, MD, PhD, Icahn School of Medicine at Mount Sinai, New York, NY
- Dax A. Hoffman, PhD, Program in Developmental Neuroscience, NICHD, Bethesda, MD
- Heidi Johansen-Berg, DPhil, University of Oxford, Oxford, UK
- Hae Ung Lee, PhD, Singapore Institute for Neurotechnology, National University of Singapore, Singapore
- Q. Richard Lu, PhD, UT Southwestern Medical Center, Dallas, TX
- Gary Lynch, PhD, University of California Irvine, Irvine, CA
- Klaus-Armin Nave, PhD, Max Planck Institute for Experimental Medicine, Göttingen, Germany
- Maiken Nedergaard, MD, DMSc, University of Rochester Medical Center, Rochester, NY
- Paul Nunez, PhD, Tulane University, New Orleans, LA
- Fernando Ortiz, PhD, Université René Descartes, Paris, France
- Ray Perez, PhD, Office of Naval Research, Arlington, VA
- Rea Raven, PhD, NICHD
- Terrence Sejnowski, PhD, Howard Hughes Medical Institute, Salk Institute, La Jolla, CA
- Beth Stevens, PhD, Boston Children's Hospital, Harvard University, Boston, MA
- Kimberly Sullivan, PhD, Boston University School of Public Health, Boston, MA
- Hiroaki Wake, PhD, National Institute for Basic Biology, Okazaki, Japan
- Robert Zatorre, PhD, Montreal Neurological Institute, McGill University, Montreal, Canada
- Joshua Zimmerberg, PhD, Program in Physical Biology, NICHD, Bethesda, MD
Contact
For more information, email fieldsd@mail.nih.gov or visit nsdps.nichd.nih.gov