You are here: Home > Unit on Neuronal Connectivity
Development and Function of Drosophila Visual Circuits
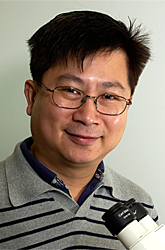
- Chi-Hon Lee, MD, PhD, Head, Unit on Neuronal Connectivity
- Songling Huang, PhD, Postdoctoral Fellow
- Moyi Li, BA, Biological Laboratory Technician
- Tzu-Yang Lin, PhD, Postdoctoral Fellow
- Chun-Yuan Ting, PhD, Research Fellow
We use the Drosophila visual system as a model to study two key questions in neurobiology: (1) how neurons form complex synaptic connections during development; and (2) how the assembled neuronal circuits function to guide animal behaviors. The fly retina contains three types of photoreceptors, R1-6, R7, and R8, each responding to a specific spectrum of light and projecting axons, in retinotopic fashion, to a specific layer in the brain. Retinotopic map formation and layer-specific targeting are the most general organizing features of complex brains in vertebrates and invertebrates. To study visual circuit assembly, we undertook forward genetic approaches to identify genes involved in the formation of proper connections between R7 and their target neurons in the brain. Our current study focuses on the TGF-beta/activin signaling pathway, which regulates retinotopic map formation and synaptogenesis of R7s. To study the function of vision circuits, we are dissecting the synaptic circuits that mediate motion detection and color vision. We have identified the neurons that serve as synaptic targets for photoreceptors and determined their functional requirement for various visual-driven behaviors.
Molecular mechanisms regulating synaptic target selection of R7 photoreceptor axons
In the fly visual system, the UV-responsive R7 photoreceptor neurons connect to the M6 layer in the medulla neuropil, where they synapse with Dm8 amacrine neurons and Tm5 projection neurons. In addition, these connections form a retinotopic map: each R7 axon innervates a single medulla column while preserving neighbor relationships in the eye. Layer-specific connectivity and retinotopic map are the characteristic features of all complex visual systems. Using a forward genetic approach in Drosophila, we are studying how layer-specific connections and the retinotopic map are established during development. We have identified adhesive molecules (such as N-cadherin) and signaling receptors (such as receptor tyrosine phosphatases) that function in R7 photoreceptors to regulate layer-specific targeting. Our investigation of the functions of these molecules strongly supports the notion that R7 growth cones are steered to the correct target layer by regulating actin cytoskeleton and adhesive interactions. In contrast, much less is known about how R7 growth cones, once they reach the correct target layer, form synapses with specific target neurons within their retinotopically correct columns.
We and others have previously shown that the retinotopic map in flies is formed in two separate stages. In the larval stage, a coarse topographic map is established, at least in part, by the secreted protein DWnt4—expressed in the target region—and its receptor Dfrizzled2 on photoreceptor axons. In past years, we studied how the R7 retinotopic map is maintained and further refined at the pupal stages. In a genetic screen based on visual-driven behaviors, we identified pex (premature extension), which affects the refinement of the R7 retinotopic map. Pex is a temperature-sensitive allele of baboon, which encodes a type I TGF-beta/activin receptor. Baboon-mutant R7 axons target to the appropriate retinotopic medulla column in the correct layer but extend collaterals to innervate neighboring medulla columns, indicating that map formation, but not layer-specific targeting, is disrupted. In addition, the presynaptic structure of R7s, as visualized by tagged-synaptotagmin, is disrupted in baboon mutants, suggesting that activin signaling plays a role in synaptogenesis.
We further showed that the other known components of the canonical activin signaling pathway are required in R7s for retinotopic map formation. Mutations disrupting dSmad2, which encodes the transcription factor downstream of baboon, result in baboon-like R7 phenotypes. In addition, we identified Importin-alpha3 as a new component of the activin signaling pathway. Importin-alpha3 mutants exhibit R7 retinotopic map defects essentially identical to those in baboon/dSmad2 mutants. Importin-alpha3 and dSmad2 are present in the R7 growth cones and form physical complexes. Removing Importin-alpha3 in R7s disrupts normal nuclear accumulation of dSmad2, suggesting that Importin-alpha3 is required for nuclear import of dSmad2. While activin is expressed in both R7s and subsets of medulla neurons, disrupting activin function solely in R7s—using RNAi or a dominant negative construct—results in baboon-like R7 phenotypes. Together, these data suggest that autocrine activin refines the R7 retinotopic map through baboon and dSmad2/importin-alpha3 complexes.
We recently discovered that activin acts not only on R7s (as an autocrine effector) but also on Dm8s, synaptic target neurons (as a paracrine effector) of R7s. Mutant Dm8 neurons devoid of activin signaling were found to have overgrowing dendritic arbors that failed to form synaptic contact with R7s. Based on these observations, we hypothesize that photoreceptor-derived activin is delivered to and activated at the R7-recipient layer to coordinate the development of pre- and post-synaptic partners and to promote mutual synaptogenesis.
Mapping color-vision circuits
We study color vision because color is in some ways the most salient visual attribute. Flies offer abundant genetic tools for visualizing the connection patterns as well as for manipulating neural activity and many visual-driven behaviors to gain functional readout. Similar to the vertebrate retina, fly visual circuits contain a large number of neuron subtypes forming complex interconnections. Because of this complexity, we applied a “divide and conquer” strategy. First, we identify key neuron subtypes and their synaptic partners based on their unique axonal and dendritic patterns. Second, we subdivide these neurons into several subclasses based on their use of specific neurotransmitters and receptors and on other gene expression patterns. Finally, we determine the neurons' functions by inactivating or restoring their synaptic function and examining the behavioral consequences. This allows us to assign specific functions to different neuron subtypes, therefore establishing causality.
Our current study focuses on determining the identify and function of the second/third-order interneurons, which synapse with R7 (UV channel), R8 (green/blue channel), or lamina neurons (the achromatic channel). Using the promoter of the histamine-gated chloride channels, we identified three synaptic partners of R7 and R8. These include Tm5 and Tm9, two types of projection neurons, which receive direct synaptic inputs from R7 and R8, respectively, as well as the L3 lamina neurons. These projection neurons likely serve as color opponent neurons and relay spectral information to the higher visual center, the lobula. In addition, we found an amacrine neuron type—Dm8—which receives input from multiple R7 inputs and relays them to Tm5. These synaptic connections have been further confirmed by serial electron-microscopic reconstruction. The core color-vision circuit, thus comprises the photoreceptors, one lamina neuron type, two projection neuron types and one amacrine neuron type. The overall architecture of the core color-vision circuit in Drosophila resembles that of vertebrates.
To determine the function of specific subtypes of the first-order interneurons, we selectively inactivated or restored the synaptic function of distinct neuron subtypes and examined the behavioral consequences. This approach requires expressing transgenes specifically in single neuron subtypes. To do so, we developed a new combinatorial gene expression system called split-LexA, which operates in parallel to the existing split-Gal4 system. Using these two systems, we were able to manipulate the activity of distinct neuron subtypes and test whether a specific neuron subtype is “required” or “sufficient” for a certain visual function. We found that inactivating the synaptic activity of the Dm8 amacrine neuron disrupted UV-preference in color-discriminating behavior tests but not motion detection, suggesting that Dm8s are specifically required for color vision. Conversely, inactivating both L1 and L2 lamina neurons abolished the animals’ ability to respond to motion stimuli while their color-discrimination ability remained intact, indicating that the lamina neurons L1 and L2 are specifically required for motion detection. Similarly, rescuing the expression of the histamine chloride channel Ort in Dm8s in an ort-mutant background completely restored UV preference, indicating that the synaptic path from R7 to Dm8, then to Tm5, is sufficient for detecting dim UV light. Rescuing the expression of the histamine chloride channel Ort in either L1 or L2 in the ort-mutant background completely restored motion detection but not color discrimination, indicating that L1 and L2 function redundantly in motion detection. In summary, our study reveals that specific visual functions can be assigned to distinct neuron subtypes and that color and motion pathways are largely segregated in the early visual pathways.
Publications
- Gao S, Takemura S-Y, Ting C-Y Huang S, Lu Z, Luan H, Rister J, Thum AS, YangM, Hong S-T, Wang JW, Odenwald WF, White BH, Meinertzhagen IA, Lee C-H. The neural substrate of spectral preference in Drosophila. Neuron 2008 60:328-342.
- Root CM, Masuyama K, Green DS, Enell LE, Nassel DR, Lee C-H, Wang JW. A presynaptic gaincontrol mechanism fine-tunes olfactory behavior. Neuron 2008 59:311-321.
- Meinertzhagen IA, Takemura SY, Lu Z, Huang S, Gao S, Ting C-Y, Lee C-H. From form to function: the ways to know a neuron. J Neurogenet 2009 23:68-77.
- Hsu S-N, Yonekura S, Ting C-Y, Robertson HM, Iwai Y, Uemura T, Lee C-H, Chiba A. Conserved alternative splicing and expression patterns of arthropod N-cadherin. PLoS Genet 2009 5:e1000441.
Collaborators
- Ian Meinertzhagen, PhD, DSc, Dalhousie University, Halifax, Canada
- Thomas Pohida, MSEE, Division of Computational Bioscience, CIT, NIH, Bethesda, MD
- Randy Pursley, MSEE, Division of Computational Bioscience, CIT, NIH, Bethesda, MD
- Mihaela Serpe, PhD, Program in Cellular Regulation and Metabolism, NICHD, Bethesda, MD
- Paul Smith, PhD, Laboratory of Bioengineering and Physical Science, NIBIB, NIH, Bethesda, MD
- Mark Stopfer, PhD, Program in Developmental Neuroscience, NICHD, Bethesda, MD
- Jing Wang, PhD, University of California San Diego, La Jolla, CA
- Benjamin White, PhD, Laboratory of Molecular Biology, NIMH, Bethesda, MD