Structural and Chemical Biology
- Anirban Banerjee,
PhD, Head, Section on Structural and Chemical Biology - Elliot Murphy, PhD, Postdoctoral Intramural Research Training Award Fellow
- Hyojung Kim, PhD, Visiting Fellow
- Chandrashekhar Kumar, PhD, Visiting Fellow
- Tanmay Mondal, PhD, Visiting Fellow
- Rahul Raina, PhD, Visiting Fellow
- Ateek Shah, PhD, Visiting Fellow
- Dawson Hillyer, BS, Postbaccalaureate Fellow
- Christian Knott, BS, Postbaccalaureate Fellow
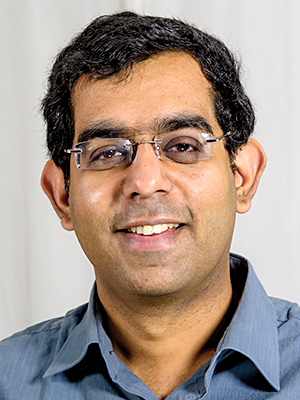
Molecular mechanism of post-translational protein S-acylation and deacylation
Co- and post-translational modifications greatly expand the structural, chemical, and functional diversity of the proteome. Of these, protein lipidation, which collectively refers to covalent modification of proteins by lipids, constitutes a centrally important class of post-translational modification. Protein S-acylation, commonly known as protein palmitoylation, is a specific form of protein lipidation whereby long-chain fatty acids, typically C16 palmitic acid, become covalently attached to cytosol-facing cysteines through a thioester linkage. S-acylation is one of the most pervasive and physiologically important post-translational modifications, with thousands of substrates ranging from ion channels to cell-surface receptors, neuronal scaffolding proteins, and small GTPases. The physico-chemical effect of S-acylation is to alter the local hydrophobicity of the substrate protein. The thioester bond makes S-acylation unique in that it is a labile moiety and can be cleaved, in the cellular context, by thioesterase enzymes, which makes S-acylation one of the few dynamic post-translational modifications and unique among different forms of protein lipidation. The physiological effects of S-acylation are diverse and are of critical cellular importance. For example, Ras, a small GTPase that is critical for cellular growth and differentiation and is mutated in about one-third of all human cancers, is S-acylated at the Golgi and subsequently targeted to the plasma membrane by vesicular transport. S-acylated Ras localizes to cholesterol-rich domains on the plasma membrane. However, it is subsequently deacylated by the thioesterase APT1, dissociates from the plasma membrane, and redistributes on endomembranes, including the Golgi. Such dynamic recycling of Ras is critical for its function. On the other hand, in recent work we showed that the Spike protein of SARS-CoV-2, the causative agent of COVID-19, is S-acylated, which is important in the viral life cycle.
Protein S-acylation is catalyzed by a large group of enzymes known as zDHHC palmitoyl acyltransferases (also referred to as DHHC enzymes or DHHC-PAT), so named because they contain a signature D-H-H-C motif (aspartate-histidine-histidine-cysteine) in a cysteine-rich domain (CRD) in an intracellular loop (Figure 1). These are low-abundance, polytopic, integral membrane proteins localized to a variety of cellular compartments. Humans have 23 zDHHC proteins encoded in their genome. Beyond the shared DHHC domain, zDHHC proteins vary considerably; some possess ankyrin repeats (structural protein motifs that mediate protein-protein interactions), a few have six transmembrane helices instead of the usual four, and at least one forms a functional heterodimer with a cytoplasmic auxiliary subunit. To date, no consensus sequence has been identified for protein S-acylation. A specific zDHHC enzyme can S-acylate many substrates, and, conversely, a given substrate can be S-acylated by many zDHHC enzymes. Such redundancy has been one of the most intriguing aspects of protein S-acylation and makes it difficult to assign substrates by overexpression/knockout strategies, given that, in the absence of one zDHHC enzyme, other zDHHC family members can take over. However, this does not necessarily reflect the true enzyme-substrate relationship. The problem is even more confounded by the lack of specific inhibitors of zDHHC enzymes. Even though 2-bromopalmitate is widely used as a global inhibitor of zDHHC enzymes, it has been shown that it also broadly targets other proteins involved in lipid metabolism.
Figure 1. Organization and properties of DHHC palmitoyltransferases (PATs)
A. The organization of three different DHHC-PATs is shown schematically. The spheres indicate protein-protein interaction domains. Erf2 associates with a cytoplasmic subunit, Erf4, to form the active enzyme.
B. The DHHC-CRD (cysteine-rich domain) region of a few representative DHHC-PATs are aligned. The conserved amino acids are shown in red.
C. Reaction catalyzed by DHHC-PATs; the reverse reaction is catalyzed by acylprotein thioesterases (APT).
Figure 1. Organization and properties of DHHC palmitoyltransferases (PATs)
A. The organization of three different DHHC-PATs is shown schematically. The spheres indicate protein-protein interaction domains. Erf2 associates with a cytoplasmic subunit, Erf4, to form the active enzyme.
B. The DHHC-CRD (cysteine-rich domain) region of a few representative DHHC-PATs are aligned. The conserved amino acids are shown in red.
C. Reaction catalyzed by DHHC-PATs; the reverse reaction is catalyzed by acylprotein thioesterases (APT).
Besides its broad importance in cell biology, S-acylation has been linked to several diseases, most notably neuro-psychiatric disorders such as Huntington’s disease and various forms of cancer. Recently, it was shown that zDHHC20 palmitoylates epidermal growth factor (EGFR) and is thus a potential therapeutic target in a wide range of cancers. More recently, zDHHC3 has been proposed as a target for cancer treatment owing to its activity as the palmitoyltransferase for the programmed cell-death ligand 1 (PD-L1). However, despite their importance across a broad spectrum of biological pathways and their biomedical importance, nothing was known about the structural mechanism of zDHHC S-acyltransferases when we started working on this family.
We leveraged the unique benefits of the NIH intramural program and started from scratch working on the structures of these eukaryotic integral membrane proteins. We earlier reported the first high-resolution crystal structures of two members of the zDHHC family: human zDHHC20 (hDHHC20) and zebrafish zDHHC15 (Figure 2a). They reveal a tepee-like transmembrane-domain organization, which splays apart towards the cytoplasmic side and harbors the active site at the membrane-aqueous interfacial region (Figure 2b), thus readily explaining why membrane-proximal cysteines are palmitoylated. We also solved the structure of hDHHC20 irreversibly modified by a covalent inhibitor, 2-bromopalmitate. The structure mimics the auto-acylated intermediate state in the enzymatic pathway and thus reveals how the acyl group of fatty acyl–CoA binds in a cavity formed in the bilayer by the transmembrane domain (Figure 2c). Residues lining the cavity contact the acyl chain, and their mutation affects enzymatic activity. By mutating two residues at the tapering end of the cavity, we also showed that we can change the acyl-chain-length selectivity of the mutant enzymes (Figure 2d). Thus, the cavity functions as a molecular ruler in determining the acyl-chain length selectivity of hDHHC20, which is important because, although palmitate is the most prevalent fatty acid used by DHHC palmitoyltransferases, they can use fatty acyl–CoAs of other chain lengths, a property that varies between different members of the DHHC family. These studies were a major advancement in the field and was used by many other labs as the starting point of their studies.
Figure 2. Structure, function, and membrane deformation of DHHC palmitoyltransferases
A. The structure of human DHHC20 and a catalytically inactive mutant of zebrafish DHHC15 shown in ribbon trace: the transmembrane domain (TM) is shown in green, the DHHC–containing cysteine-rich domain in blue and the C-terminal domain in orange; the grey spheres indicate Zn2+ ions; these are both Golgi-resident enzymes, and thus the top side faces the Golgi lumen and the active site the cytoplasm.
B. Active site of human DHHC20 showing the catalytic triad containing the active-site cysteine.
C. Structure of human DHHC20 irreversibly modified with 2-bromopalmitate, which results in the active-site cysteine linking to the alpha-carbon of palmitic acid (the acyl group of palmitic acid is shown in stick rendition); also shown are two residues towards the top of the tapering cavity to where the palmitate binds.
D. The acyl chain–length selectivity of wild-type (WT) human DHHC20: mutation of tyrosine181 to alanine (Y181A) expands the cavity and shifts the acyl selectivity to the longer side; on the other hand, mutation of serine29 to phenylalanine (S29F) contracts the cavity and thus shifts the acyl selectivity to the shorter side.
Figure 2. Structure, function, and membrane deformation of DHHC palmitoyltransferases
A. The structure of human DHHC20 and a catalytically inactive mutant of zebrafish DHHC15 shown in ribbon trace: the transmembrane domain (TM) is shown in green, the DHHC–containing cysteine-rich domain in blue and the C-terminal domain in orange; the grey spheres indicate Zn2+ ions; these are both Golgi-resident enzymes, and thus the top side faces the Golgi lumen and the active site the cytoplasm.
B. Active site of human DHHC20 showing the catalytic triad containing the active-site cysteine.
C. Structure of human DHHC20 irreversibly modified with 2-bromopalmitate, which results in the active-site cysteine linking to the alpha-carbon of palmitic acid (the acyl group of palmitic acid is shown in stick rendition); also shown are two residues towards the top of the tapering cavity to where the palmitate binds.
D. The acyl chain–length selectivity of wild-type (WT) human DHHC20: mutation of tyrosine181 to alanine (Y181A) expands the cavity and shifts the acyl selectivity to the longer side; on the other hand, mutation of serine29 to phenylalanine (S29F) contracts the cavity and thus shifts the acyl selectivity to the shorter side.
An outstanding question in the field of protein S-acylation is the nature of zDHHC-substrate interactions, which has remained poorly understood for most zDHHC enzymes. Cell-based experiments indicate a promiscuous and complex zDHHC–substrate network, whereas lack of in vitro reconstitution experiments has impeded insights into the nature of discrete zDHHC–substrate interactions. We designed a novel substrate S-acylation reconstitution assay called the Pep-PAT assay, using purified enzyme and peptide fragments of substrates (Figure 3). We use the Pep-PAT assay to investigate the substrate S-acylation of three different zDHHC enzymes on seven different substrates. Remarkably, all the zDHHC enzymes showed robust activity with certain substrates but not others (Figure 3). These in vitro reconstitution experiments indicate that there is a preferred substrate hierarchy for zDHHC enzymes. We further used the Pep-PAT assay to interrogate the role of neighboring residues around the target cysteine on S-acylation of PSD-95 (postsynaptic density protein 95) and SARS-CoV-2 Spike protein. Select residues around the target cysteines have a distinct impact on substrate S-acylation, leading to the first insights into how neighboring residues around the target cysteine affect substrate S-acylation by zDHHC enzymes. We also used in cellulo assays to validate the impact of neighboring residues on substrate S-acylation. We were the first to report reconstituted substrate selectivity of zDHHC enzymes, and they build a framework for understanding the interactions between zDHHC enzymes and their substrates.
Figure 3. Pep-PAT assay for in vitro reconstitution of the substrate selectivity of zDHHC enzymes
A. A substrate S-acylation assay, which we call the Pep-PAT assay, using purified zDHHC enzymes and synthetic peptide fragments of substrates and coupling the released CoASH (coenzyme A) with a second enzyme to generate stoichiometric amount of NADH, which is fluorescent.
B. The design of the substrate fragments involve attachment of an N-terminal myristoyl moiety to mimic the local hydrophobic environment of the target cysteine.
C. Pep-PAT assay showing that human zDHHC20 has robust activity on SARS-CoV-2 Spike protein but not PSD-95 (postsynaptic density protein 95).
D. Pep-PAT assay showing that human zDHHC15 has robust activity on the same PSD-95 substrate as in C.
Figure 3. Pep-PAT assay for in vitro reconstitution of the substrate selectivity of zDHHC enzymes
A. A substrate S-acylation assay, which we call the Pep-PAT assay, using purified zDHHC enzymes and synthetic peptide fragments of substrates and coupling the released CoASH (coenzyme A) with a second enzyme to generate stoichiometric amount of NADH, which is fluorescent.
B. The design of the substrate fragments involve attachment of an N-terminal myristoyl moiety to mimic the local hydrophobic environment of the target cysteine.
C. Pep-PAT assay showing that human zDHHC20 has robust activity on SARS-CoV-2 Spike protein but not PSD-95 (postsynaptic density protein 95).
D. Pep-PAT assay showing that human zDHHC15 has robust activity on the same PSD-95 substrate as in C.
Protein S-acylation is reversed by the action of protein thioesterases, that then constitute the “erasers” of protein S-acylation. Yet, they are even more poorly understood than zDHHC enzymes, which catalyze the attachment of the fatty acids. Until relatively recently, only two protein thioesterases were known, i.e., APT1 and APT2. Members of the ABHD (α/β-hydrolase domain) family of serine hydrolyses have more recently been discovered to catalyze the deacylation of numerous protein substrates. Of them, the most prominent substrates are the four isoforms of Ras that are mutated in many human cancers. The S-acylation/deacylation cycle of NRas and other Ras family members plays pivotal roles in the regulation of their subcellular localization. It was recently discovered that members of the ABHD17 family of depalmitoylases are the key enzymes that regulate localization of NRas by removing palmitate from its C-terminus. Yet, there is still no direct evidence that ABHD17 is indeed an NRas depalmitoylase. We purified ABHD17 and demonstrated that it is a bona fide NRas depalmitoylase using an in vitro biochemical assay. We first established a method for producing C-terminally processed, dually lipidated NRas, which is, to the best of our knowledge, the first purification of full-length NRas, opening new avenues for investigating the molecular mechanism of the oncoprotein. We identified the catalytic triad of residues in ABHD17 and show that a highly conserved loop in ABHD17 plays an important role in its NRas depalmitoylation. Our study illuminates the importance of both catalytic activity and spatial co-localization with their substrates as an important determinant of the cellular activity of membrane peripheral enzymes and thus, a putative mode of their regulation. It also paves the way for a comprehensive molecular understanding of both NRas as well as its depalmitoylase ABHD17 and sets up a platform for the discovery of honed small molecule inhibitors as promising leads for drug discovery.
Figure 4. In vitro reconstitution of NRas depalmitoylation by human ABHD17
A. Size-exclusion chromatography of recombinant ABHD17.
B. SDS-PAGE analysis of fractions containing ABHD17.
C. Click chemistry–based assay investigating the palmitoylation of NRas and the action of added ABHD17, as monitored by the alkyne analog of palmitate on NRas and visualized by click chemistry–based attachment of a Rhodamine fluorophore. The Coomassie-stained gel shows loading levels of NRas.
Figure 4. In vitro reconstitution of NRas depalmitoylation by human ABHD17
A. Size-exclusion chromatography of recombinant ABHD17.
B. SDS-PAGE analysis of fractions containing ABHD17.
C. Click chemistry–based assay investigating the palmitoylation of NRas and the action of added ABHD17, as monitored by the alkyne analog of palmitate on NRas and visualized by click chemistry–based attachment of a Rhodamine fluorophore. The Coomassie-stained gel shows loading levels of NRas.
Molecular mechanism of iron and polyamine transport across cellular membranes
The importance of iron in biology cannot be overstated. In higher organisms, mitochondria are the ‘hotspot’ for the cell biology of iron, because, there, Fe-S clusters are biosynthesized and iron is inserted into heme. Mitochondrial iron homeostasis plays a critical role in cellular iron homeostasis and in the overall physiology of the cell. In vertebrates, the only known major transporters of iron into mitochondria are mitoferrin-1 and mitoferrin-2, two homologous members of a large group of mitochondrial transporters known as the Mitochondrial Carrier family (Figure 5). Mitoferrin-1 (Mfrn1) is expressed mainly in erythroid cells, while mitoferrin-2 (Mfrn2) is expressed ubiquitously. Knockout of Mfrn1 is embryonically lethal, reflecting the importance of mitoferrins in vertebrate physiology.
Mfrn1 and Mfrn2 were discovered more than 15 years ago. However, the proposed iron-transport activity had not been demonstrated using an in vitro functional reconstitution assay, and nothing was known about their interaction with iron or other related metal ions, most likely because heterologous overexpression and purification of mitoferrins were not reported in the literature. There were also no reports of a reconstituted iron-transport assay starting from purified protein. In earlier work, we carried out heterologous purification, in vitro functional reconstitution, and mutational dissection of a vertebrate Mfrn1, the first demonstration that Mfrn1 can indeed transport iron. Our studies provided the first biochemical insights into Mfrn function. In earlier work, we also used our in vitro proteoliposome-reconstituted iron-transport assay, the first such assay to be reported in the literature, to dissect the iron-transport activity of MavN, another highly conserved iron transporter, in the bacterial pathogen Legionella pneumophila (Figure 5b). We are currently working towards understanding the detailed mechanism of iron recognition and transport by Mfrn1 and MavN.
Figure 5. Transporters of iron and polyamine
A. Iron is imported through the plasma membrane by the transferrin/transferrin receptor (blue) cycle and is transported out of endosomes by the divalent metal ion transporter (DMT) (grey); iron is delivered to mitoferrin (yellow cylinders) by unknown means; mitoferrin delivers iron to unknown partners in mitochondria, which become available for heme and Fe-S cluster biosynthesis.
B. Schematic showing Legionella entering a host cell and sequestering itself in a Legionella-containing vacuole (LCV): MavN is inserted into the membrane of the LCV and hijacks iron from the host cell.
Figure 5. Transporters of iron and polyamine
A. Iron is imported through the plasma membrane by the transferrin/transferrin receptor (blue) cycle and is transported out of endosomes by the divalent metal ion transporter (DMT) (grey); iron is delivered to mitoferrin (yellow cylinders) by unknown means; mitoferrin delivers iron to unknown partners in mitochondria, which become available for heme and Fe-S cluster biosynthesis.
B. Schematic showing Legionella entering a host cell and sequestering itself in a Legionella-containing vacuole (LCV): MavN is inserted into the membrane of the LCV and hijacks iron from the host cell.
Additional Funding
- Office of Dietary Supplements
Publications
- ROS-dependent palmitoylation is an obligate licensing modification for GSDMD pore formation. Nature 2024 630:437–446
- In vitro reconstitution of transition metal transporters. J Biol Chem 2024 300:107589
Collaborators
- Mark Distefano, PhD, University of Minnesota, Minneapolis, MN
- Rodolfo Ghirlando, PhD, Laboratory of Molecular Biology, NIDDK, Bethesda, MD
- Ralph Isberg, PhD, Tufts University School of Medicine, Boston, MA
- Rolf Swenson, PhD, The Chemistry and Synthesis Center, NHLBI, Rockville, MD
Contact
For more information, email anirban.banerjee@nih.gov or visit https://www.nichd.nih.gov/research/atNICHD/Investigators/banerjee.