RNA Metabolism in Cell Biology, Growth, and Development
- Richard J. Maraia,
MD, Head, Section on Molecular and Cellular Biology - Sergei Gaidamakov, PhD, Biologist
- Sandy Mattijssen, PhD, Staff Scientist
- Alex Vassilev, PhD, Staff Scientist
- Alan Kessler, PhD, Postdoctoral Fellow
- Ian Schowe, BS, Postbaccalaureate Intramural Research Training Award Fellow
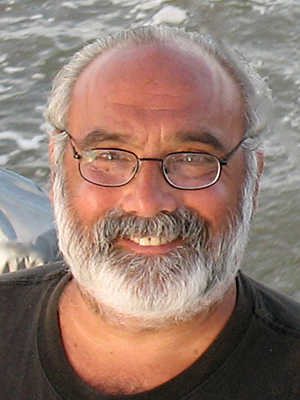
We study the biology and relevance to human health of mRNAs and tRNAs, the principal molecular messengers and adapter agents used to decipher and express our genetic code. The biogenesis, processing, modification, and decay of tRNAs and mRNAs, and isoforms and fragments thereof, as well as some of their specific interacting proteins that contribute to cell proliferation, growth, and development during health and disease, are the topics of our experimental investigations and other studies. Some studies focus on the La protein, which interacts with nuclear precursor tRNAs and the few other newly transcribed short transcripts synthesized by RNA polymerase III (Pol III), which are matured to small noncoding (nc) RNAs of diverse critical functions. The La protein is a unique chaperone for Pol III transcripts. It binds to and protects their shared 3′-oligo(U) motifs from exonucleolytic digestion and decay, can assist proper folding, and for many, retains them in the nucleus to acquire specific modifications and undergo specific RNA–processing steps prior to export into the cytoplasm. Surprisingly, accumulating evidence suggests that failure to produce properly matured Pol III ncRNAs can contribute to disease states independent of the requirement for normal processes, but also because they can activate cellular innate immune response and other surveillance pathways [reviewed in Reference 5]. Other studies focus on the La–related proteins (LARPs) 1 and 4, which share similar activities for RNA 3′ end binding and protection from exonucleolytic digestion, but for cytoplasmic mRNAs rather than for nuclear precursor ncRNAs. Related to mature functional tRNAs and their role in mRNA translation, we focus on the tRNA anticodon-loop modification enzyme known as tRNA-isopentenyltransferase-1 (TRIT1). The La protein is also referred to as "La antigen" for its long-known involvement as a target of auto-antibodies in patients with chronic inflammatory autoimmune disorders such as systemic lupus erythematosus (SLE), Sjögren’s syndrome (SS), and neonatal lupus. The official name for the gene encoding La protein is SSB (Sjögren’s syndrome antigen-B).
tRNAs are produced at over 10-fold higher molar equivalents than ribosomes during cellular proliferation. More than 500 unique human tRNA gene loci comprise the largest subset of genes actively transcribed by Pol III, followed by 15–30 nearly identical copies of 5S ribosomal RNA genes and several additional unique “single copy” ncRNA genes. The La protein serves to promote overall proper tRNA maturation. Many diseases are attributable to defective tRNA biogenesis and the consequent failure to support mRNA translation. Related to surveillance, Pol III itself acts directly in a dedicated innate immune activation pathway separate from its nuclear transcription role. In addition, a few Pol III–transcribed ncRNAs appear to have also been co-opted by the innate immune system [Reference 5]. Recent findings in our lab extend this potential to Pol III transcripts of a minority subset of (human) tRNA genes that can activate innate immune and inflammatory pathways. Further, this work indicates that the La protein can suppress the innate immune and inflammatory activity of these tRNA gene transcripts in human cells [Kessler et al, under revision].
Pol III synthesizes high levels of tRNA by a conserved process of transcription termination–associated re-initiation, relevant to development and cancer. Termination occurs within a short tract of T residues in the non-template (NT) DNA strand at the ends of Pol III–transcribed genes. The nascent transcripts bear a copy of this terminator, U(n)U-3′OH, a recognition motif for the nuclear La protein, which binds in a sequence- and length-dependent manner. Notably, the 3′U(n) length–dependence of La binding is usually shorter than the minimal T-length required for efficient Pol III termination, the latter of which is 6, 5, and 4 Ts for Saccharomyces cerevisiae, Schizosaccharomyces pombe, and humans, respectively. This suggests an La link with tRNA expression, a link that fits with human Pol III, which evolved a minimal 4T termination mechanism; the data suggest that this may direct some post-transcriptional events. Studies on development, structure, and its gene variants indicate that human Pol III evolved termination mechanisms to control gene-regulatory programs with greater intricacy.
The human La protein is a target of auto-antibodies in patients with chronic inflammatory diseases such as systemic lupus erythematosus, Sjögren’s syndrome (SS) (La is also known as SS antigen-B, SSB), and neonatal lupus. Although La is active in other immune pathways, evidence for its primary involvement in autoimmunity is lacking. As noted above, La binds to POL III transcripts in all cells and serves as a chaperone during their nuclear maturation. La not only protects pre–tRNAs from 3′ exonucleases, but also directs and temporally orders the first step in the 5′ processing by the RNase P pathway. We propose a role for La in a novel pathway, different from tRNA maturation. Our data suggest that La is a determinant for a subset of pre-tRNAs (genes) to enter an alternative pathway to specific activation of the type-I interferon (IFN) response. We are studying a dual-activity tRNA gene(s) for which La is a key determinant of whether the nascent 4T–terminated transcripts are directed to tRNA maturation or to an alternate pathway of innate immune activation. Accordingly, some dual-activity tRNA genes may serve as endogenous immune adjuvants or may contribute to autoimmunity. We are currently exploring these possibilities.
The rate of RNA production by Pol III was shown to affect the efficiency by which pre-tRNAs acquire the common m2,2G26 modification, which increases tRNA activity. Disrupted tRNA biogenesis, attributable to gene variants, leads to developmental and other diseases, as well as to neurodegeneration and intellectual impairment. About 120 modifications occur on tRNAs, of which about 40 have been documented for human cytoplasmic (cy–) tRNAs and several others for human mitochondrial (mt-) tRNAs. Many tRNA–modification enzymes (TME) are multisubunit, and some composite modifications require several gene products. Thus, mutations in numerous TMEs cause disease.
Critical modifications to tRNA anticodon loops fine-tune base pairing for optimal decoding and wobble decoding of synonymous codons, which is an important component of a tunable system of high-fidelity cy–translation. It is important to note that the 61 sense codons for 20 amino acids (aa) are decoded by cy-tRNAs, which collectively carry only 45 anticodons, a feature of tRNA genes named anticodon-sparing, that is widely conserved, although the number and identity of the ‘missing’ anticodons differ among kingdoms, anti-correlated with wobble-base modifications. In humans, about 15 standard codons must be wobble-decoded by cy–tRNAs, whose activities are controlled by anticodon modifications. Thus, Pol III transcription of tRNA genes is only one level on which the translation of a range of cy–mRNAs with biased codon content can be regulated. While this constitutes a nuclear-cytoplasmic translation system with much potential for intricate regulation, the complexities of overlapping/redundant decoding activities also create potential for translation infidelity in cases of faulty modification or tRNA pool imbalance.
All TMEs are nuclear-encoded, and a minority subset modify both cy– and mt–tRNAs, with potential to synchronize translation in both compartments. Distinct decoding rules apply in mitochondria, in part because each mt–DNA encodes only one tRNA for each of 18 amino acids. Synonymous codons occupy mt–mRNAs, but are not distinguished by different tRNAs, as is the case for cy–mRNAs. For example, while four codons each for Ala, Gly, Pro, Thr, and Val are decoded by three cy–tRNAs, a single mt–tRNA for each must wobble-decode its four cognate codons. Furthermore, these mt-tRNAs use unmodified U34 as their wobble base, whereas cy–tRNA wobble bases are part of an elaborate modification system, especially for U34. Each mt–tRNA is critical; point mutations that impair mt–DNA translation are uniquely associated with oxidative-phosphorylation diseases, whereas most nuclear tRNA genes are buffered by multiple copies; a known exception is a unique, single-copy, brain-specific tRNA. Yet, some diseases caused by a mutation in a mt–tRNA exhibit a phenotype like that resulting from a nuclear gene mutation to a TME whose substrate is the mt–tRNA.
Numerous pathogenic alleles that encode defective subunits of Pol III cause hypomyelinating leukodystrophy (HLD), whose pathobiology is consistent with poor formation of axonal myelin sheaths rather than demyelination. Pol III produces several ncRNAs (non-coding RNAs) in addition to tRNAs. We suggest that another disruption mechanism of a tRNA biogenesis–CNS network is also possible. As TME defects often manifest as neurological, reflecting tissue reliance on mitochondria, and because cy– and mt–translation are synchronized, including by tRNA modifications and other factors, disruption of general tRNA homeostasis may impair mitochondrial function, with a pathophysiologic contribution to disease, including in developing oligodendrocytes.
mRNA levels are determined in significant part by levels of cognate tRNAs, from yeast to human. In addition, certain mRNAs are particularly sensitive to tRNA levels. The LARP4 mRNA has a tract of about 70 codons with a very poor match to cellular tRNA levels. Thus, unprogrammed changes in tRNA output (e.g., genetic mutation in POL III) could shift mRNA stabilities and translation efficiencies in unpredictable ways with uncertain outcomes.
Translation of LARP4 mRNA produces LARP4, which binds to poly(A) and to poly(A)–binding protein (PABP/PABPC1). LARP4 stabilizes mRNAs by opposing deadenylation of their poly(A) tails, substrates of Ccr4-Not deadenylase (a multi-protein complex that functions in gene expression in the nucleus, where it regulates transcription, and in the cytoplasm, where it associates with translating ribosomes and RNA–processing bodies). Potential for regulation is that Ccr4-Not monitors mRNA–ribosomes for codon–tRNA match. For ribosomal protein–encoding mRNAs stabilized by LARP4, this supports a working model in which LARP4 mRNA senses tRNA levels and relays this by producing LARP4 to regulate ribosome biogenesis, perhaps with LARP1.
It has been known for some time that human Pol III and associated factors are dysregulated in cancer. More recently, functionally related mRNAs favoring proliferative or differentiation states were shown to be biased in synonymous codons and in the cognate tRNAs differentially expressed in those cells. Strikingly, the first Pol III structures provide insight into activities specific to the higher eukaryotic 17-subunit complex. First, the cancer-associated RPC7a Pol III subunit paralog (encoded by POLR3G) appeared to interfere with binding of the Pol III–negative regulator and tumor suppressor MAF1, whereas the RPC7b (POLR3GL) paralog subunit is enriched in cells programmed for differentiation (limited proliferation). Yeast Pol III has only one homolog. The second example involves the most striking feature of higher eukaryote–specific Pol III: the multi-domain expansion of RPC5 and hypothesized associated higher eukaryote promoter-type specificity, and its link to termination-reinitiation recycling.
Mechanistic control of ncRNA genes appears to have evolved activities that extend human Pol III beyond the housekeeping activities of its yeast counterpart. This fits with a view of hPol III in self vs. non-self surveillance functions. Notable are Pol III Vault (Vt) ncRNAs (Vault is a hollow barrel-shaped ribonucleoprotein complex) with involvement in two activity types: innate immune surveillance, and differentiation vs. maintenance of undifferentiated states. Both Pol III Vt and snaR (small nuclear factor 90–associated RNA) ncRNAs are processed to miRNAs that exert downstream effects on mRNA profiles and/or differentiation/cancer.
Role of La-related protein-4 (LARP4) in poly(A)–mediated mRNA stabilization
Ubiquitous in eukaryotes, La proteins are involved in two broad functions: first, metabolism of a wide variety of precursor tRNAs and other small nuclear RNAs by association with these RNAs’ common UUU-3′OH–transcription termination elements; and second, translation of specific subsets of mRNAs, such as those containing 5′ IRES (internal ribosome entry site) motifs. LARP4 emerged later in evolution, and we found it to be an mRNA–associated cytoplasmic factor associated with poly(A)–binding protein C1 (PABPC1, PABP). LARP4 uses two regions to bind to PABPC1. We showed that the N-terminal domain (NTD, amino acids 1–286) of LARP4, consisting of an N-terminal region (NTR, amino acids 1–111) followed by two tandem RNA–binding motifs known as an ‘La module’ (111–285), exhibits preferential binding to poly(A). The NTR contains a unique PAM2w motif that binds to the MLLE (a peptide-binding domain) of PABP. The group of our collaborator Maria Conte showed that the NTR itself is responsible for most of the poly(A) binding and that, moreover, this involves conserved residues unique to the PAM2w of LARP4. The La module is flanked by a different motif, each independently interacting with PABP. LARP4 is controlled at the level of mRNA stability: one level of control is by an A+U-rich element (ARE) in its 3′ UTR via interactions with the protein tristetraproline (TTP), the latter of which is regulated in mammals by tumor necrosis factor alpha (TNFα); a second level of control was found for the LARP4 mRNA–coding sequence in an unusual group of synonymous codons with poor match to cellular tRNA levels [Reference 1]. The LARP4 protein controls the metabolism/homeostasis and translation of heterologous mRNAs by affecting their poly(A) tail length. Working with researchers in the NICHD Molecular Genomics Core facility, we developed a single-molecule, high-throughput nucleotide-resolution poly(A)–tail sequencing method, referred to as SM-PAT-seq, which yielded insights into LARP4 function and mechanism. LARP4 is a global factor involved in mRNA poly(A) length homeostasis and appears to effect mRNA stabilization by opposing the action of deadenylases when poly(A) tails are short.
Activities of eukaryotic RNA polymerase III (Pol III) and associated factors
The Pol III multisubunit enzyme complex consists of 17 integral subunits, whereas Pols I and II consist of 14 and 12 respectively. However, during early vertebrate evolution, one of the Pol III subunits, POLR3G/RPC7, was duplicated, and both gene paralogs evolved as essential, such that present-day organisms use both. Thus, each molecule of cellular Pol III contains one or the other paralog, i.e., POLR3G or POLRGL, and this subunit can control Pol III–intrinsic activity and to direct cellular pathways toward differentiation-quiescence or proliferation-cancer type phenotypes.
The transcription factor TFIIIC, composed of six subunits, binds to A- and B-box promoters (promoter elements of tRNA genes) and recruits TFIIIB to direct Pol III to the correct start site. TFIIIB–Pol III complexes appear highly stable and demonstrate great productivity in supporting the many cycles of initiation, termination, and re-initiation necessary to produce the more than tenfold molar excess of tRNAs relative to ribosomes, which is required to drive translation during growth and development. In contrast to all other multisubunit RNA polymerases, termination and re-initiation by Pol III are functionally, if not physically, linked. POLR3G and POLRGL can control the extent to which Pol III may be sensitive to inhibition by the Pol III–negative regulator Maf1 and to recycling. Our laboratory developed in vivo and biochemical methods to examine the unique mechanisms used by Pol III. Hereditary mutations in Pol III cause hypomyelinating leukodystrophy (HLD), as well as defects in innate immunity. In addition to its being essential for cell proliferation, Pol III is also linked to aging.
Transcription termination delineates 3′ ends of gene transcripts, prevents otherwise runaway Pol from intruding into downstream genes and regulatory elements, and enables release of the Pol for recycling. While other Pols require complex cis signals and/or accessory factors to accomplish these activities, eukaryotic Pol III does so autonomously with high efficiency and precision at a simple oligo(dT) stretch of 5–6 base pairs. A basis for this high-density cis information is that both the template and non-template strands of the Pol III terminator carry distinct signals for different stages of termination. High-density cis information is a feature of the Pol III system, which is also reflected in the dual functionalities of the tRNA promoters as both DNA and RNA elements. Furthermore, the TFIIF–like Pol III subunit C37 is required for this function of the non-template strand signal. Our results reveal the Pol III terminator to be an information-rich control element. While the template strand promotes destabilization via a weak oligo(rU:dA) hybrid, the non-template strand provides distinct sequence-specific destabilizing information through interactions with the C37 subunit.
Control of the differential abundance or activity of tRNAs can be an important determinant of gene regulation. Pol III synthesizes all tRNAs in eukaryotes, and its derepression is associated with cancer. MAF1 is a conserved general repressor of Pol III under the control of TOR (target of rapamycin), which acts to integrate transcriptional output and protein-synthetic demand toward metabolic economy. We used tRNA–Hydro-seq (hydrolysis-based tRNA sequencing) to document that little change occurred in the relative levels of different tRNAs in maf1–mutated cells. By contrast, the efficiency of N2,N2-dimethyl G26 (m2,2G26) modification on certain tRNAs was reduced in response to maf1 deletion and associated with anti-suppression, which we validated by other methods. Overexpression of Trm1 (tRNA dimethyl transferase), which produces m2,2G26, reversed maf1 anti-suppression. The model that emerges is that competition by elevated tRNA levels in maf1-delta cells leads to m2,2G26 hypo-modification resulting from limiting Trm1, thus reducing the activity of suppressor tRNASerUCA (UCA is the anticodon for serine) and accounting for anti-suppression. Consistent with this, Pol III mutations associated with HLD reduce tRNA transcription, increase m2,2G26 efficiency, and reverse anti-suppression. Extending this more broadly, a reduction in tRNA synthesis by treatment with rapamycin leads to increased m2,2G26 modification, a response that is conserved among highly divergent yeasts and human cells [Arimbasseri AG et al, PLoS Genetics 2015;11:e1005671].
The ability of Pol III to efficiently recycle from termination to re-initiation is critical for abundant tRNA production during cellular proliferation, development, and cancer. We used two tRNA–mediated suppression systems to screen for Rpc1 (subunit of Pol III) mutants with gain- and loss-of-termination phenotypes in the yeast S. pombe. We mapped 122 point mutation mutants to a recently solved 3.9 Å structure of the yeast Pol III elongation complex (EC); they cluster in the active-center bridge helix and trigger loop, as well as in the pore and funnel formation, the latter indicating involvement in termination of the RNA–cleavage domain of the C11 subunit of Pol III. Biochemical, kinetic, and genetic data indicate that mutants with the RT (readthrough) phenotype synthesize more RNA than wild-type cells and, surprisingly, more than can be accounted for by the mutants' increased elongation rate. Importantly, similar mutations in spontaneous cancer suggest this is an unforeseen mechanism of Pol III activation in disease.
Fission yeast as a model system for the study of tRNA metabolism and function in translation
More than 23 years ago, we began developing, refining, and advancing a tRNA–mediated suppression (TMS) system in the fission yeast Schizosaccharomyces pombe, which provides a ‘red-white’ phenotypic real-time assay that can be used to investigate various aspects of tRNA biogenesis, maturation, and metabolism in vivo. In S. pombe, the human La protein can replace the tRNA–processing/maturation function of Sla1p, the S. pombe equivalent of the La protein. Moreover, in S. pombe, human La is faithfully phosphorylated on Ser-366 by the protein kinase CKII, the same enzyme that phosphorylates Ser-366 in human cells, and this phosphorylation event promotes pre-tRNA processing. We are using the system to study transcription by RNAP III, post-transcriptional processing, and tRNA modifications by conserved enzymes that produce tRNA isopentenyl-adenosine-37 and dimethyl-guanosine-26 (N2,N2-dimethyl G26, m2,2G26).
tRNAs, codon use, and mRNA metabolism in growth and development
A major interest of ours is to decipher what we refer to as ‘secondary information’ in the genetic code, information that is derived from mRNAs' biased use of synonymous codons. This can produce a layer of information beyond the amino acid sequence of a protein; i.e., in addition to providing the template for the sequence of a protein, the use of certain synonymous codons can also produce additional biochemical effects, which we refer to as ‘secondary information.’ The effects can be related to ribosome pausing, which can affect protein folding, or to alterations in the stability of the mRNA. Other types of secondary information can also be encoded in synonymous codons; for example, sets of mRNAs that share similar patterns of synonymous codon bias are similarly sensitive to tRNAs with the same anticodon modification and exhibit similar patterns of efficiency of translation elongation. The components of the secondary information system are the tRNA pool, the tRNA–modification enzymes, and the codon-bias distribution among the mRNAs. We recently found that synonymous codon use by the human LARP4 mRNA is a key determinant in the control of the expression levels of its mRNA and protein, and that increases in otherwise limiting tRNAs, that are cognate to these codons, increase LARP4 production, which in turn activates LARP4, to promote a net increase in the poly(A) tail length of heterologous mRNAs, including those that encode ribosomal protein subunits [Reference 1], which may be important because ribosome production is regulated during growth and development, and because the potential circuit involving LARP4 control by tRNA could be an important point of control.
Publications
- The short conserved region-2 of LARP4 interacts with ribosome-associated RACK1 and promotes translation. Nucleic Acids Res 2025 53(3)gkaf053
- Single-Molecule Poly(A) Tail Sequencing (SM-PATseq) Using the PacBio Platform. Methods Mol Biol 2024 2723:285–301
- Cell surface-bound La protein regulates the cell fusion stage of osteoclastogenesis. Nat Commun 2023 14:616
- Structural basis of 3’-end poly(A) RNA recognition by LARP1. Nucleic Acids Res 2022 50:9534–9547
- The nuclear and cytoplasmic activities of RNA polymerase III, and an evolving transcriptome for surveillance. Nucleic Acids Res 2021 49(21):12017–12034
- Mechanism of RNA polymerase III termination-associated reinitiation-recycling conferred by the essential function of the N terminal-and-linker domain of the C11 subunit. Nat Commun 2021 12:5900
Collaborators
- Maria R. Conte, PhD, King's College, University of London, London, United Kingdom
- Ryan Dale, MS, PhD, Bioinformatics and Scientific Programming Core, NICHD, Bethesda, MD
- Markus Hafner, PhD, RNA Molecular Biology Group, NIAMS
- James R. Iben, PhD, Molecular Genomics Core, NICHD, Bethesda, MD
- Gennady H. Margolin, PhD, Bioinformatics and Scientific Programming Core, NICHD, Bethesda, MD
- Eugene Valkov, DPhil, RNA Biology Laboratory, Center for Cancer Research, NCI, Frederick, MD
Contact
For more information, email maraiar@mail.nih.gov or visit https://www.nichd.nih.gov/research/atNICHD/Investigators/maraia.