Mechanisms Regulating Fate and Maturation of Forebrain GABAergic Interneurons
- Timothy J. Petros,
PhD, Head, Unit on Cellular and Molecular Neurodevelopment - Yajun Zhang, BM, Biologist
- Arushi Chauhan, PhD, Postdoctoral Fellow
- Jianing Li, PhD, Postdoctoral Fellow
- Matthew Manion, PhD, Postdoctoral Fellow
- Samra Beyene, MS, Postbaccalaureate Fellow
- Vedant Garg, BS, Postbaccalaureate Fellow
- Anthony Tanzillo, BS, Postbaccalaureate Fellow
- Allison Tucker, BS, Postbaccalaureate Fellow
- Aidan Johantges, Summer Intern
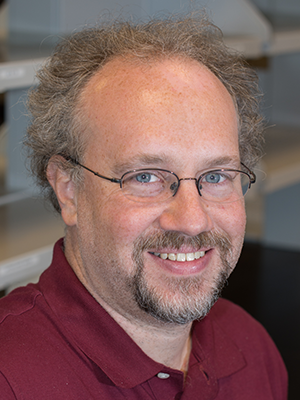
Proper brain function requires a balance between excitatory projection neurons and GABAergic inhibitory interneurons. Although interneurons constitute the minority (about 20%) of neurons in the brain, they are the primary source of inhibition and are critical components in the modulation and refinement of the flow of information throughout the nervous system. Interneurons are an extremely heterogeneous cell population, with distinct morphologies, connectivity, neurochemical markers, and electrophysiological properties. In fact, the incredible diversity and heterogeneity of interneurons was observed over a century ago, with Ramón y Cajal hypothesizing in Recollections of My Life that “The functional superiority of the human brain is intimately linked up with the prodigious abundance and unaccustomed wealth of the so-called neurons with short axons.” Abnormal development and function of interneurons has been linked to the pathobiology of numerous neurological and psychiatric diseases, such as epilepsy, schizophrenia, and autism. Many genes implicated in brain disorders are enriched in young interneurons, and thus a thorough description of the cellular and molecular mechanisms regulating this diverse cell population is necessary to understand both normal development and disease models.
The lab focuses on understanding the how intrinsic genetic and epigenetic programs interact with the local brain environmental to generate this incredible diversity of interneuron subtypes during normal development and in disease models (Figure 1). We take a multifaceted approach to this issue, utilizing both in vitro and in vivo approaches to identify candidate mechanisms that regulate interneuron fate decisions. We strive to develop cutting-edge techniques that will overcome the many challenges of studying interneuron development. Our ultimate goal is to discover genetic cascades and signaling mechanisms that direct interneuron differentiation and maturation during normal development and in disease states.
Figure 1. MGE–derived GABAergic cells populate many different brain regions.
The image depicts a section of an embryonic brain (left) that has been electroplated to label cells derived from the medial ganglionic eminence (MGE), merged with a section of an adult brain (right), displaying the incredible spatial and morphological diversity of MGE–derived cells in the mature brain. Understanding how this heterogeneous population is generated from one embryonic brain structure is the focus of this laboratory.
Figure 1. MGE–derived GABAergic cells populate many different brain regions.
The image depicts a section of an embryonic brain (left) that has been electroplated to label cells derived from the medial ganglionic eminence (MGE), merged with a section of an adult brain (right), displaying the incredible spatial and morphological diversity of MGE–derived cells in the mature brain. Understanding how this heterogeneous population is generated from one embryonic brain structure is the focus of this laboratory.
Loss of Ezh2 in the medial ganglionic eminence (MGE) alters interneuron fate and function.
We were interested in exploring how genetic perturbations alter normal processes and regulate interneuron fate. There is growing evidence that mutations in epigenetic machinery can lead to neuro-development disorders. One gene of interest was Enhancer of zeste homolog 2 (EZH2), which encodes the primary methyltransferase of the polycomb repressive complex 2 (PRC2), an enzyme that is critical for trimethylation of histone 3 lysine 27 (H3K27me3), resulting in gene repression. In humans, EZH2 variants lead to the Weaver syndrome, a complex disease with varying degrees of intellectual disability. Loss of Ezh2 in mice can lead to premature neuronal differentiation, migration defects, and changes in neuronal fate, but the role of Ezh2 in forebrain interneurons had not been explored. To this end, we generated Nkx2.1-Cre;Ezh2 conditional knockout (KO) mice to remove Ezh2 from the MGE.
Loss of Ezh2 reduced the number of MGE–derived interneurons in the cortex and caused a shift in fate, with an increase in SST+ (somatostatin-positive) and a reduction in PV+ (parvalbumin-positive) interneurons (Figure 2A). Similar shifts in cell fate were observed in the hippocampus and striatum. To remove Ezh2 specifically from postmitotic cells, we used used a Dlx6a-Cre transgenic mouse line to remove Ezh2 only in postmitotic interneuron progenitors. We did not observe altered cell fate in these mice, indicating that the critical function of Ezh2 occurs in cycling neural progenitors. In collaboration with Soohyun Lee, we characterized the electrophysiological (e-phys) properties of mutant cells in the cortex. We found no changes in the intrinsic e-phys properties of PV+ or SST+ cells in the cortex of KO mice. However, PV+ cells in the KO mice displayed more complex axonal arbors, with greater axonal length and arborization than in wild-type (WT) PV+ cells (Figure 2B). This could be a form of compensation, given that one way to circumvent the loss of PV+ cells in the mutant would be for the surviving cells to have increased synaptic outputs and increased inhibition.
Figure 2. Loss of Ezh2 in the MGE affects interneuron fate and function.
A. Ezh2 KO mice have a reduction of MGE–derived cortical interneurons, with increased SST+ and decreased PV+ cells.
B. Fast-spiking PV+ cells display more complex axonal arbors in Ezh2 KO mice.
C. Top, Volcano plot showing genes enriched in SST+ cells (SST, Pde1a) in Ezh2 KO MGE and genes enriched in PV+ cells (Mef2c, Maf) in the WT MGE. Bottom, corresponding UMAP plots for SST, Mef2c, and Maf.
D. Top, MA plot showing genomic loci differential changes in H3K27me3 levels in the Ezh2 KO mouse, as determined by CUT&TAG. Bottom, Trace showing H3K27me3 levels at genes more susceptible (Foxp4) or resistant (Nkx2.1) to loss of Ezh2.
Figure 2. Loss of Ezh2 in the MGE affects interneuron fate and function.
A. Ezh2 KO mice have a reduction of MGE–derived cortical interneurons, with increased SST+ and decreased PV+ cells.
B. Fast-spiking PV+ cells display more complex axonal arbors in Ezh2 KO mice.
C. Top, Volcano plot showing genes enriched in SST+ cells (SST, Pde1a) in Ezh2 KO MGE and genes enriched in PV+ cells (Mef2c, Maf) in the WT MGE. Bottom, corresponding UMAP plots for SST, Mef2c, and Maf.
D. Top, MA plot showing genomic loci differential changes in H3K27me3 levels in the Ezh2 KO mouse, as determined by CUT&TAG. Bottom, Trace showing H3K27me3 levels at genes more susceptible (Foxp4) or resistant (Nkx2.1) to loss of Ezh2.
We performed the 10x Genomics Multiome assay (snRNA-seq and snATAC-seq) on the MGE of WT and KO mice. We found an increase in genes expressed by SST+ cells (SST, Pde1a) in the KO MGE, whereas genes predictive of PV–fated interneurons (Mef2c, Maf) were enriched in WT MGE (Figure 2C). We also observed concurrent shifts in the presence of Mef2c– and Maf–binding motifs in accessible regions in the snATAC-seq data. Thus, loss of Ezh2 induces transcriptional and epigenetic changes in the embryonic MGE, which lead to shifts in mature interneuron fate. We also quantified changes in H3K27me3 levels at specific genomic loci via CUT&Tag. Despite a global reduction in H3K27me3 in Ezh2 KO mice, we observed relative changes in H3K27me3 at specific loci. For example, H3K27me3 signal was nearly entirely depleted at the Foxp4 locus, indicating that this region is extremely susceptible to loss of Ezh2. Conversely, the Nkx2.1 locus displayed a relative increase in H3K27me3, meaning that it was significantly resistance to this epigenetic perturbation (Figure 2D). While the function of Foxp4 in interneuron development unclear, Nkx2.1 is a ‘master regulator’ of MGE–derived interneurons. The finding implies that genes critical for specific aspects of development (such as Nkx2.1 in the MGE) may be evolutionarily resistant to epigenetic perturbations [Reference 6].
Disruption of gene activation in the MGE induces drastic cellular and behavioral phenotypes.
While the previous study exploring Ezh2 function addressed how perturbation of gene repression changed interneuron fate, alteration of gene activation is also associated with a variety of neurodevelopmental disorders. Dysregulation of H3K4 methylation, a critical step in gene activation, is associated with numerous neural developmental disorders, autism spectrum disorder, schizophrenia, and substance-related disorders (Figure 3A). Despite its importance, studying H3K4 has been challenging because it can be methylated by six different proteins, and experimentally deleting a single methyltransferase can lead to confounding compensations by other proteins (Figure 3B). We devised a creative solution to this long-standing problem by utilizing a Cre–dependent transgenic mouse in which the H3K4 lysine is mutated to a methionine (H3K4M), thus blocking methylation at this residue in any protein. Given that this is a transgenic mouse line, the homozygous H3K4M mouse also provides a considerable advantage: with two WT H3K4 alleles and two mutant H3K4M alleles, the mouse is a functional heterozygote and thus a good model for exploring the role of altered H3K4 methylation in human disease.
Our lab generated Nkx2.1-Cre;H3K4M homozygous (Hom) mice to target MGE cells (Figure 3C) and found that such H3K4M Hom mice display a complex phenotype with a wide variety of deficits. Half of the mutant mice died within the first 5 months, and survivors were significantly smaller in body weight and brain size than littermates. However, the Hom mice later become obese, which is likely the result of gene activation changes in the hypothalamus, as Nkx2.1 is also expressed in this region (Figure 3E–F). H3K4M Hom mice also suffered spontaneous seizures and increased seizure susceptibility, caused by the significant reduction of inhibitory interneurons in the cortex and hippocampus (Figure 3D). Further, MGE–derived interneurons in Hom mice displayed greater variability in their intrinsic electrophysiological properties in ways that mimicked immature interneurons. We performed a comprehensive battery of behavioral tests, revealing that the H3K4M Hom mice displayed reduced ambulatory locomotion, increased anxiety-like behaviors, altered gait, and deficits in learning and memory (Figure 3G–H). Many of these phenotypes are consistent with the neural developmental disorders observed in patients with alterations of H3K4 methylation. Additionally, single-cell Multiome analysis revealed distinct gene-expression profiles and cellular trajectories of WT and Hom cells in postmitotic neurons in the MGE and hypothalamus. A manuscript describing this study is in preparation.
Figure 3. Disruption of H3K4 methylation in the MGE
A. Role of H3K4 methylation in disease.
B. Genes regulating H3K4 methylation.
C. H3.3.K4M transgenic mouse (84).
D. Decrease of MGE–derived cortical interneurons in Nkx2.1Cre;H3K4M mouse.
E–F. K4M Hom mice are smaller in the first postnatal weeks (E) but then become obese after 5 months (F).
G. K4M Hom mice display decreased ambulatory movement in home cage.
H. K4M Hom mice display increased anxiety and locomotion in open field test.
Figure 3. Disruption of H3K4 methylation in the MGE
A. Role of H3K4 methylation in disease.
B. Genes regulating H3K4 methylation.
C. H3.3.K4M transgenic mouse (84).
D. Decrease of MGE–derived cortical interneurons in Nkx2.1Cre;H3K4M mouse.
E–F. K4M Hom mice are smaller in the first postnatal weeks (E) but then become obese after 5 months (F).
G. K4M Hom mice display decreased ambulatory movement in home cage.
H. K4M Hom mice display increased anxiety and locomotion in open field test.
Perturbing chromatin architecture disrupts gene expression in the MGE.
Characterizing how chromatin architecture regulates proper promoter-enhancer interactions and gene expression is critical to an understanding of normal development and disease etiologies. Many promoter-enhancer interactions are tissue- or cell-type–specific, so that it is critical to study these interactions in vivo. Our previous study identified a direct chromatin interaction between Nkx2.1 and a gene upstream called MAP3K12 binding inhibitory protein 1 (MBIP). Like Nkx2.1, MBIP is also only expressed in the MGE [Rhodes CT et al. Nat Commun 2022;13:419] (Figure 4A). Given that Nkx2.1 is a ‘master regulator’ of MGE–derived interneurons, Nkx2.1 represents an ideal locus to explore how brain region–specific chromatin interactions regulate gene expression and cell fate.
We used a cutting-edge genetic strategy to generate a mouse line that disrupts the MGE–specific Nkx2.1-Mbip interaction, as confirmed by Micro-C (Nkx2.1–CTCF mice, Figure 4B). There is a strong reduction of Mbip and a moderate downregulation of Nkx2.1 in the MGE in these mice (Figure 4C). We are currently performing the following set of experiments on Nkx2.1–CTCF (CTCF is a transcriptional repressor) mice. First, we will examine Nkx2.1 and Mbip expression in the MGE at different ages, as well as other MGE–enriched genes. Second, we will perform single-cell Multiome sequencing and CUT&Tag on H3K4me3 (active promoter) and H3K27me3 (active enhancer) to characterize changes in the transcriptome, chromatin accessibility and histone modifications in the MGE of Nkx2.1–CTCF mutant mice. Third, we will count the number of SST+ and PV+ neurons in the cortex to determine whether there are any changes in the density and/or proportion of MGE–derived interneurons in the Nkx2.1–CTCF mutant mice. Success with this project will motivate us to apply these genetic perturbations to other genomic loci at critical fate-determining genes that have brain region–specific chromatin interactions.
Figure 4. Perturbation of chromatin interaction linking Nkx2.1 and MBIP
A. MGE–specific interaction between Nkx2.1 and Mbip (black arrowheads).
B. 6 CTCF sites were inserted between Nkx2-1 and Mbip to block this interaction (top). Micro-C confirmed loss of this Nkx2.1-Mbip interaction in the MGE (black arrowheads) with a corresponding new interaction at the ectopic CTCF sites (purple arrowheads).
C. Strong decrease of Mbip mRNA and moderate reduction of Nkx2.1 mRNA in the MGE of Nkx2.1-CTCF mice.
Figure 4. Perturbation of chromatin interaction linking Nkx2.1 and MBIP
A. MGE–specific interaction between Nkx2.1 and Mbip (black arrowheads).
B. 6 CTCF sites were inserted between Nkx2-1 and Mbip to block this interaction (top). Micro-C confirmed loss of this Nkx2.1-Mbip interaction in the MGE (black arrowheads) with a corresponding new interaction at the ectopic CTCF sites (purple arrowheads).
C. Strong decrease of Mbip mRNA and moderate reduction of Nkx2.1 mRNA in the MGE of Nkx2.1-CTCF mice.
Mechanisms regulating fate determination of caudal ganglionic eminence (CGE)–derived interneurons
While significant progress has been made characterizing mechanisms regulating initial fate decisions of MGE–derived interneurons, our understanding of CGE–derived interneurons lags significantly behind. This is in part because we lack genetic tools to specifically target and manipulate CGE–derived cells. The CGE contributes about 30% of interneurons in mice, but humans and primates have a larger proportion of CGE–derived interneurons. Additionally, CGE progenitors are the primary source of tumors and cortical lesions in the tuberous sclerosis complex (TSC), underscoring the importance of understanding development of CGE cells.
Previous studies transplanted fluorescent embryonic precursors from MGE subdomains into postnatal brains to determine the spatial and temporal origin of mature interneuron subtypes. These studies identified a spatial and temporal organization relating MGE progenitors to mature interneuron subtypes (Figure 5A–B). Combining these insights with gene-expression patterns has generated important insights into MGE development. Surprisingly, whether a similar organization occurs in the CGE has not been explored. To this end, we are harvesting anterior, posterior, ventral, and dorsal CGE cells (aCGE, pCGE, vCGE, dCGE) from Dlx6aCre;Ai9 mice (in which all cells fluoresce red) and transplanting these cells into the cortex of WT pups (Figure 5C–E). We are harvesting CGE subdomains from two different ages (E13.5 and E15.5) to identify both temporal and spatial relationships between CGE progenitors and mature interneuron subtypes, as both location and birthdate play a role in fate determination within the MGE. In total, this constitutes eight different conditions (four CGE subdomains at two different time points). Brains are harvested at P30–35, sectioned and immunostained for CGE–specific interneuron markers (e.g., VIP, CCK, reelin, and calretinin) to correlate spatial and temporal origin with mature CGE–derived interneuron subtypes (Figure 5F). Our preliminary cell counts from aCGE and pCGE transplants indicate that a spatial bias does exist in the CGE, with distinct CGE–derived interneuron subtypes preferentially arising from specific CGE subdomains. Our CGE spatio-temporal map will be integrated with our scRNA-seq datasets described above to identify candidate genes expressed in CGE subdomains related to mature subtypes (Figure 5G). We expect to publish a manuscript with these results in the coming year.
Figure 5. Cell transplants to determine the spatial-temporal relationship between CGE subdomains and mature interneuron fates
A–B. Spatial organization (A) and temporal birthdate (B) relating MGE progenitors to mature subtypes.
C. Dissection to harvest anterior (A) and posterior (P) CGE.
D. Injection setup.
E. Schematic of CGE cell injection.
F. P21 brain that was transplanted at P1 with E13.5 CGE cells from Dlx6aCre;Ai9 mouse displaying VIP+/Tom+ (arrows) and Reelin+/Tom+ (arrowheads) cells.
G. Schematic of possible results to integrate with our previous scRNA-Seq data.
Figure 5. Cell transplants to determine the spatial-temporal relationship between CGE subdomains and mature interneuron fates
A–B. Spatial organization (A) and temporal birthdate (B) relating MGE progenitors to mature subtypes.
C. Dissection to harvest anterior (A) and posterior (P) CGE.
D. Injection setup.
E. Schematic of CGE cell injection.
F. P21 brain that was transplanted at P1 with E13.5 CGE cells from Dlx6aCre;Ai9 mouse displaying VIP+/Tom+ (arrows) and Reelin+/Tom+ (arrowheads) cells.
G. Schematic of possible results to integrate with our previous scRNA-Seq data.
Additional Funding
- NICHD Scientific Director's Award (2023–2024), concluded
Publications
- Loss of Ezh2 in the medial ganglionic eminence alters interneuron fate, cell morphology and gene expression profiles. Front Cell Neurosci 2024 18:1334244
- Generation of single cell and single nuclei suspensions from embryonic and adult mouse brains. STAR Protocols 2023 4(1):1–22
- Enhancers that activate target genes across CTCF boundaries increase phenotypic robustness. Nat Genet 2023 55(2):280–290
- The association of GNB5 with Alzheimer disease revealed by genomic analysis restricted to variants impacting gene function. Am J Hum Genet 2024 111(3):473–486
- Structural perturbation of chromatin domains with multiple developmental regulators can severely impact gene regulation and development. bioRxiv 2024 1–23
- Loss of Ezh2 in the medial ganglionic eminence alters interneuron fate, cell morphology and gene expression profiles. Front Cell Neurosci 2024 18:1334244
Collaborators
- Susan Amara, PhD, Laboratory of Molecular and Cellular Neurobiology, NIMH, Bethesda, MD
- Ryan Dale, PhD, Bioinformatics & Scientific Programming Core, NICHD, Bethesda, MD
- Soohyun Lee, PhD, Unit on Functional Neural Circuits, NIMH, Bethesda, MD
- Chris McBain, PhD, Section on Cellular and Synaptic Physiology, NICHD, Bethesda, MD
- Pedro Rocha, PhD, Unit on Genome Structure and Regulation, NICHD, Bethesda, MD
- Mark Stopfer, PhD, Section on Sensory Coding and Neural Ensembles, NICHD, Bethesda, MD
- Benjamin White, PhD, Section on Neural Function, NIMH, Bethesda, MD
Contact
For more information, email tim.petros@nih.gov or visit https://www.nichd.nih.gov/research/atNICHD/Investigators/petros.