You are here: Home > Section on Medical Biophysics
Medical Biophysics
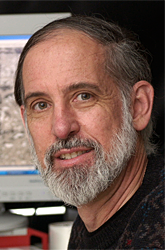
- Robert F. Bonner, PhD, Head, Section on Medical Biophysics
- Martin Ehler, PhD, Visiting Fellow
- Emily J. King, PhD, Postdoctoral Fellow
- Kimberly Tran, MS, Technical Training Fellow
- Zigurts Majumdar, PhD, Research Fellow
- Sanford Meyers, MD, Guest Researcher
We focus on developing new optical technologies that better characterize or modify early stressors that drive chronic diseases and could be useful in developing effective disease prevention strategies. We continue to develop our inventions of expression microdissection and laser capture microdissection for better integration with multiplex molecular analysis of specific cells and organelles extracted from complex tissue.
Through refinements in automated analysis of integrated clinical multispectral, multimodal retinal image sets, we seek to map the in vivo distribution and dynamics of several naturally occurring photochemicals implicated in health preservation and early disease. In clinical studies, we apply our new technology approaches to determine how changes in retinal spectral irradiance affect the balance between retinal photochemical pathways that may drive early, more readily reversible disease. Our broader goals are to better quantify local molecular imbalances within the retina and to develop reliable means for classifying and quantifying early disease in order to evaluate the effectiveness of strategies to prevent disease progression.
Laser microdissection and molecular diagnostics technology development
Integrative molecular biology requires an understanding of interactions of large numbers of pathways. Similarly, molecular medicine increasingly relies on complex macromolecular diagnostics to guide therapeutic choices. A fundamental argument for laser capture microdissection (LCM) of tissues is that, without separation of specific cell populations from complex tissues, we would miss critical control functions of thousands of regulated transcription factors, cell regulators, and receptors that are expressed at low copy number. By detecting changes in these critical effectors, we expect to improve our integrative understanding of tissue function and pathology. In complex tissues—particularly among pathological variations—it is exceptionally difficult to measure the majority of molecules that are at low copy number per cell without first isolating specific cell populations. The LCM techniques that we developed are now widely used in molecular analysis of genetics and gene expression changes in target cells within complex tissues.
In global proteomic and lipid studies without molecular amplification methods, the quantity of isolated cells sufficient to perform accurate characterization of less abundant species is problematic because the microscopic visualization, targeting, and isolation in LCM has a maximal rate of 1–20 cells per second, depending on the cells' microscopic distribution within the tissues. Recently, in collaboration with NCI and CIT, we invented (US Patents #7,709,047 and #7,695,752) and are now refining xMD, an automatic "target-directed microtransfer" technique based on macromolecule-specific staining of specific proteins or organelles. Combining rapid laser scanning and such specific absorptive stains, we automatically microbond tissue targets as small as 1 micron to our thermoplastic transfer films while scanning about 100,000 cells per sec. This conceptually simple device requires neither microscopes nor user targeting decisions to harvest specifically stained targets directly from sections of complex tissues. The spatial resolution is determined by stain localization (less than 1 µ) and molecular polymer bonds, greatly exceeding the optical diffraction limits of the laser scanner. Consequently, it is uniquely suited to isolating highly dispersed, specific cell populations (e.g., stem cells or specific neural subpopulations in the brain) or even specific organelles. As in LCM, the spatial relationships among the microbonded targets in the tissue are preserved on the transfer film. Recently, with funding from a NIH Director's Challenge Grant, we joined a multi-institute team (NICHD, NIMH, NIBIB, NCI, and CIT) to refine our xMD prototype for proteomic analysis of specific organelles within tissue sections.
Gene expression during normal development and pathology progression
If microdissection and molecular analysis can be made clinically practical, the expression levels of sets of approximately 20–100 critical, stage-specific disease markers within a selected cell population might provide reliable diagnosis and intermediate endpoints of response to molecular therapies in individual patients. Our analysis of large gene-expression and protein databases suggests that a significant fraction of all genes is expressed in any specific cell type and that the levels of gene products universally exhibit a highly skewed power-law distribution similar to those characterizing many other complex systems (Kuznetsov et al., Genetics 2002;161:1321). In collaboration with Jacob Brown and Brian Brooks, we devised a method for using LCM to integrate small regions from the developing eye around the time of topological closure in the mouse embryo for comprehensive gene expression analysis in order to identify candidate genes associated with coloboma, a common human development defect. In collaboration with NCI and the University of Maryland, we are developing novel nonlinear clustering and dimensionality reduction algorithms to identify temporal classes within microarray gene expression data from LCM-dissected target tissue. We have evaluated the extracted relationships among clusters of genes with lower expression levels, using statistical analysis of associated gene pathways (GoMiner) for the development of integrative methods to study normal development and function in tissues.
Our research in statistics of less abundant gene products and suitable detection methods using microdissection is guided by a vision of molecular diagnosis evolving from one based on qualitative or quantitative analysis of a few key macromolecules to one in which dimensionality reduction and classification algorithms analyze complex multivariate databases. Such analysis could allow a more complete identification of highly correlated clinical cases and the characterization of their response to molecular therapies specifically designed to prevent progression.
Prevention of progression of age-related macular degeneration through photoprotection
In our clinical collaborations at the NIH and with the National Naval Medical Research Center, the Walter Reed Army Medical Center, and the Institute of Eye Diseases in Moscow, we are seeking to apply non-invasive spectral imaging methods to study early photochemical injury and the means of limiting it. Lipofuscin accumulates with age in the retinal pigment epithelium (RPE) and contains undigestible fluorescent photoproducts of the A2E pathway, which are the dominant visible-light fluorophores in the retina. Fluorescent lipofuscin colocalizes with the acute injury caused by photosensitization of reactive oxygen intermediates (ROI) in the primate retina when exposed to unnaturally high doses of blue light. This observation has led to a widely held hypothesis that cumulative blue-light photochemistry is harmful and plays a role in AMD progression, in spite of epidemiological studies that suggest, at most, small effects of cumulative sunlight exposure and cataract surgery. We developed a biophysical model to quantitatively relate age-dependent changes in human retinal spectral irradiance at all ambient light levels to levels of bisretinoid accumulation and their photochemical production of ROI within the RPE. ROI levels in the RPE generated by blue light–induced lipofuscin photochemistry decrease significantly with aging but increase almost an order of magnitude following cataract surgery, according to our model. These findings are inconsistent with the hypothesis that blue light–induced ROI production in the RPE is a significant early driver of age-related macular degeneration.
Our alternative hypothesis is that the critical early stressor in AMD and Stargardt's macular dystrophy is the increased RPE levels of unoxidized bisretinoids resulting from an imbalance in their rates of photochemical production within the photoreceptors (e.g., green light in the rods) and their rates of photochemical oxidation within the RPE lipofuscin by violet-blue light. The bisretinoid precursors (principally A2PEH2) of A2E and its oxidation products that segregate within lipofuscin granules in the RPE originate from reactions of all-trans-retinal within the photoreceptor outer segments (ROS) when in bright daylight. Although RPE lysosomal processing enzymatically digests over 99% of shed ROS contents, A2E and related fluorophores are not enzymatically broken down but rather concentrate into lipofuscin granules. Our theory posits that further processing of bisretinoids within the RPE requires their photo-oxidation by short wavelength violet-blue light. In younger years, higher cumulative retinal exposures that produce unoxidized bisretinoids at higher rates contain enough violet-blue to photochemically oxidize A2E sufficiently to keep the steady state levels constant. This spectral balance between photochemical production of the primary bisretinoids and their further photochemical processing is slowly lost as the lens ages. By age 60, the average steady-state concentrations within the RPE lipofuscin reach 200 µM in normal eyes. However, A2E is toxic to cellular membranes at much lower concentrations. Our biophysical model quantitatively demonstrates how the natural yellowing of the human lens with aging can reduce the relative rate of photo-oxidation of accumulating bisretinoids within the RPE secondary lysosomes and lead to increased levels of unoxidized cytotoxic retinoids. If our hypothesis is correct, external spectral filtering (e.g. rod-protecting, vermilion sunglasses) could dramatically lower rates of bisretinoid production and reduce levels of our presumed RPE stressors driving age-related retinal pathology.
Our clinical multispectral autofluorescence imaging studies are designed to test our biophysical model and clinical hypothesis that spectral irradiance changes drive age-related changes within the bisretinoid pathway and that spectral sunglasses can reduce levels of toxic photochemicals within the retinal pigment epithelium. By further refining multispectral autofluorescence imaging, using both confocal scanning laser ophthalmoscopes and fundus cameras, we are trying to map changes in the retinal distributions of the various fluorescent bisretinoids that can be changed by altered retinal spectral irradiance. We specifically want to detect predicted changes in bisretinoid fluorescent species following 1) cataract surgery and IOL implantation and 2) wearing bicolored spectral sunglasses when in bright daylight. We are currently refining autofluorescence imaging protocols and multispectral image analysis in order to provide high-resolution, molecularly specific images of early microscopic changes and to follow their changes over time. To this end we have, with NSF support, established a multi-institution effort to develop more effective quantitative image analysis methods capable of characterizing microscopic retinal changes associated with the earliest disease states. In collaboration with clinicians in NEI's Clinical Branch, we are developing and testing new quantitative tools for following non-invasively the earliest changes in macular pathology in AMD and other retinal diseases. Our goal is to provide better early diagnosis and methods to evaluate early intervention and disease prevention strategies. If our spectral imbalance hypothesis is correct, this process should be reversible during the earliest stages of retinal pathology. Our multispectral autofluorescence imaging could provide a means to evaluate such prevention strategies by quantitatively following preclinical microscopic changes within the retina noninvasively.
Temporal-spectral programmable LED lighting to optimize health
Modern life is associated with 24-7 activities made possible by ubiquitous uses of artificial lighting. Nonvisual retinal photoreception via blue-light (460-480nm peak) activation of melanopsin within a subset of retinal ganglion cells has demonstrated a critical role for average blue-light irradiance levels on attention and synchronization of circadian physiology. Human evolution has developed such optimized controls based on normal variations in retinal irradiance associated with the diurnal solar cycle. Modern life and ubiquitous artificial light sources have greatly modified temporal patterns in retinal irradiance, which may have such diverse health effects as increased incidence of cancer, metabolic syndrome, sleep disturbances, and attention deficit and depressive disorders. Conversely natural seasonal variations in daylight periods can induce seasonal-affected disorder, which responds to early morning artificial blue-light enrichment. Advances in more energy-efficient and spectrally specific light sources such as light-emitting diodes (LEDs) offer new opportunities for optimization of the spectral composition of artificial lighting that could significantly impact biological function and health. How can we study the effects of modern lighting on health and performance in ways that might inform new lighting standards to guide commercial lighting developments?
Sunlight acting through the mc-RGC (melanopsin-containing retinal ganglion cells) pathway profoundly affects both acute brain function and daily entrainment of circadian physiology. Modern architectural design emphasizes the use of natural sunlight where possible. Night-time exposures to artificial lighting can be disruptive of circadian physiology, as shown by numerous studies on shift-workers, particularly irregular shift work. Disruption of circadian physiology appears to increase the risk for cancer, the incidence of metabolic syndrome, and possibly a wide spectrum of neuro-psychiatric disorders. As a consequence, one might improve health and productivity of our modern populace in general by optimizing spectral temporal control of artificial lighting that at night balances photopic visual acuity for night-time tasks with minimizing circadian disruption by blue-light. Conversely, enriching daytime blue light components in artificial lighting may improve acute brain functions such as alertness and prevent circadian disruptions in individuals who are indoors during most daylight hours. Activation of melanopsin in the mc-RGCs by artificial lighting that is sufficient during the daytime and minimal at night requires a new paradigm shift for the lighting industry.
Our current knowledge of human non-visual effects of spectral lighting is largely limited to isolated controlled sleep-lab studies on small numbers of subjects and risk factors in large-scale epidemiology studies. Even with significant new data about the action spectra of the mc-RGC cells (including blue-red photoreversal) and their role in important brain pathways, including acute alertness and circadian synchronization, we do not have a rational approach to designing optimal spectral lighting for health and productivity due to the difficulties in testing real-world effects of spectral temporal lighting for human performance and physiology. Common artificial light sources that contribute the highest retinal irradiance are computer monitors (also smart phones, televisions) universally designed to exceed ambient light levels reaching the retina. In our modern society, large segments of the population average four hours of computer use, and the typical household television is on for eight hours a day. These high brightness sources on which we routinely fixate for long periods are therefore most likely to alter natural patterns of activation of melanopsin-containing retinal ganglion cells and their projections to the brain. Our hypothesis is that there are significant numbers of individuals whose spectral-temporal retinal irradiance from their daily computer use (and other personal electronic devices with luminous screens) puts them at risk for circadian disruption and a variety of associated physiological stresses (e.g., contributing to the rise in metabolic syndrome). With assistance from the DOE and the Lighting Division of Lawrence Berkeley National Lab, we have developed software that controls RGB (red, green, blue) color balance of LED/LCD screen displays independently of the computer's normal operational programs. Based on published melanopsin spectral sensitivity, we can currently dynamically modulate melanopsin activation over a critical 10-fold range while maintaining photopic sensitivity and color-rendering. We are adapting this spectral-temporal testbed for its effects on acute cognitive function and alertness in a format that could be readily exported to typical office and home computer use. If we can devise reliable measures of spectral-temporal optimization, we envision developing freeware that could enable individuals to self-test and determine individual acute optimization with eventual extension to diurnal data logging for individual optimization of circadian physiology.
United States Patents
- Emmert-Buck MR, Tangrea MA, Bonner RF, Chuaqui R, Pohida TJ. Target activated microtransfer. #7,709,047; May 4, 2010.
- Bonner RF, Pohida TJ , Emmert-Buck MR, Tangrea MA, Chuaqui R,. Target activated microtransfer. #7,695,752; April 13, 2010.
- Bonner RF, Goldstein SR, Smith PD, Pohida TJ. Method of laser capture microdissection from a sample utilizing short pulse. #6,897,038; May 24, 2005.
- Liotta LA, Emmert-Buck M, Krizman DB, Chuaqui R, Linehan WM, Trent JM, Bonner RF, Goldstein SR, Smith PD, Peterson JI. Isolation of cellular material under microscopic visualization. #6,867,038; March 15, 2005.
- Bonner RF, Goldstein SR, Smith PD, Pohida TJ. Non-contact laser capture microdissection. #6,743,601; June 1, 2004.
- Goldstein SR, Bonner RF, Smith PD, Peterson J, Pohida TJ. Mechanical handling systems for laser capture microdissection. #6,720,191; April 13, 2004
- Liotta LA, Buck MF, Weiss RA, Zhuang Z, Bonner RF. Isolation of cellular material under microscopic visualization. #6,569,639; May 27, 2003.
- Bonner RF, Goldstein SR, Smith PD, Pohida TJ. Precision laser capture microdissection utilizing short pulse length. #6,420,132; July 16, 2002.
- Bonner RF, Liotta L, Buck M, Krizman DB, Chuaqui R, Linehan WM, Trent JM, Goldstein SR, Smith PD, Peterson JI. Isolation of cellular material under microscopic visualization. #6,251,516; June 26, 2001.
Additional Funding
- NSF Biophotonics 0854233 (2009-2011) Multispectral Retinal Imaging and Mapping of Naturally Occurring Fluorophore and Chromophore Distributions in Health and Early Pathology
- DOE FEMP (2010) Temporal-spectral programmable LED lighting: Test-beds for health and sustainability.
- NIH Director's Challenge Award (2009-2010) Subcellular microdissection for the identification of organelle proteins
Publications
- Brown JD, Dutta S, Bharti K, Bonner RF, Munson PJ, Dawid IB, Akhtar AL, Onojafe IF, Alur RP, Gross JM, Hejtmancik JF, Jiao X, Chan WY, Brooks BP. Expression profiling during ocular development identifies 2 Nlz genes with a critical role in optic fissure closure. Proc Natl Acad Sci USA 2009;106:1462-1467.
- Chan CC, Ross RJ, Shen DF, Ding XY, Majumdar Z, Bojanowski CM, Zhou M, Salem N, Bonner R, Tuo JS. Ccl2/Cx3cr1-deficient mice: an animal model for age-related macular degeneration. Ophthalmic Res. 2008;40:124-128.
- Wong WT, Forooghian F, Majumdar Z, Bonner RF, Cunningham D, Chew EY. Fundus autofluorescence in type 2 idiopathic macular telangiectasia: correlation with optical coherence tomography and microperimetry. Am J Ophthalmol. 2009;148:573-583.
- Ehler M, Rajapakse V, Zeeberg B, Brooks B, Brown J, Czaja W, Bonner RF. Analysis of Temporal-spatial Covariation within Gene Expression Microarray Data in an Organogenesis Model. Bioinformatics Research and Applications. LNICS 2010;6051:38-49.
Collaborators
- Brian Brooks, MD, PhD, Ophthalmic Genetics and Visual Function Branch, NEI, Bethesda, MD
- Jacob Brown, MD, PhD, Ophthalmic Genetics and Visual Function Branch, NEI, Bethesda, MD
- Emily Chew, MD, Clinical Branch, NEI, Bethesda, MD
- Denise Cuningham, CRA, RBP, MEd, Clinical Branch, NEI, Bethesda, MD
- Wojciech Czaja, PhD, Norbert Weiner Center, University of Maryland, College Park, MD
- William K. Floyd, Div Environmental Protection, OD, NIH, Bethesda, MD
- Tatiana Kiseleva, MD, DSc, Institute of Eye Diseases, Moscow, Russia
- Jennifer Lippincott-Schwartz, PhD, Cell Biology and Metabolism Program, NICHD, Bethesda, MD
- Sanford P. Markey, PhD, Laboratory of Neurotoxicology, NIMH, Bethesda, MD
- David O. Mazur, MD, Integrated Ophthalmology Service, National Naval Medical Center, Bethesda, MD
- Philip G. McQueen, PhD, Mathematical and Statistical Computing Laboratory, CIT, Bethesda, MD
- Sanford Meyers, MD, Retina Consultants, Des Plaines, IL
- Tom Pohida, MSE, Computational Biology and Electronics Laboratory, CIT, Bethesda, MD
- Wai T. Wong, MD, PhD, Ophthalmic Genetics and Visual Function Branch, NEI, Bethesda, MD
- Francis M. Rubinstein, PhD, Lawrence Berkeley National Lab, Berkeley, CA
- Barry R. Zeeberg, PhD, Laboratory of Molecular Pharmacology, NCI, Bethesda, MD
Contact
For more information, email bonnerr@mail.nih.gov.