You are here: Home > Section on Neuronal Connectivity
Assembly and Function of Chromatic Circuits in Drosophila
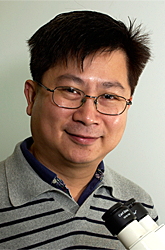
- Chi-Hon Lee, MD, PhD, Head, Section on Neuronal Connectivity
- Chun-Yuan Ting, PhD, Staff Scientist
- Thangavel Karuppudurai, PhD, Postdoctoral Fellow
- Yang Lee, PhD, Postdoctoral Fellow
- Tzu-Yang Lin, PhD, Postdoctoral Fellow
- Krishna Melnattur, PhD, Postdoctoral Fellow
- Moyi Li, BA, Biological Laboratory Technician
- Michele Rodney, BS, Postbaccalaureate Intramural Research Training Awardee
Using the Drosophila visual system as a model, we study how neurons form complex yet stereotyped synaptic connections during development and how the assembled neural circuits extract visual attributes, such as color and motion, to guide animal behaviors. Fly vision is mediated by three types of photoreceptor, R1-6, R7, and R8, each responding to a specific spectrum of light. They project their axons to three distinct layers in the brain, where the cells form synaptic connections with different target neurons. To study visual circuit functions, we combined structural and functional approaches to map visual circuits. Using both light- and electron-microscopy (EM) studies, we identified the medulla neurons that are post-synaptic to R7 and R8 photoreceptors. We determined their usage of neurotransmitters and receptors by single-cell transcript profiling. By targeted manipulation of neuronal activity, we establish the roles of these medulla neurons in processing chromatic information. For circuit development, we focus on the formation of synaptic circuits between medulla neurons and R7 and R8 photoreceptors. We use high-resolution imaging techniques and genetic manipulations to delineate the molecular mechanisms that control dendritic patterning and synaptic specificity of the medulla neurons.
Mapping color-vision circuits
Our goal is to understand how visual systems extract visual information to guide animal behaviors. We have focused on color vision in Drosophila. The tree photoreceptors R1-6, R7, and R8 connect synaptically to a distinct set of neurons in the peripheral optic lobes. We combine structural and functional approaches to map visual circuits. As stated in the introduction, light and electron-microscopy identified the medulla neurons that are post-synaptic to the chromatic photoreceptors R7 and R8 and likely process color information. By systematically inactivating or restoring the functions of specific neuronal types and examining behavioral consequences, we assigned the functions of the medulla neurons in true color vision and innate spectral preference. By RNAi–mediated knock-down of synaptic components and functional imaging, we are determining the synaptic mechanisms that process color information.
Color vision, which differentiates spectral compositions independently of brightness, provides animals, from insects to primates, with great power for object recognition and memory registration and retrieval. Using a combination of genetic, histological, electrophysiological, and behavioral approaches, we study how the visual system processes chromatic information to guide behaviors in Drosophila. Using color-preference and color-learning assays, we demonstrated that flies innately prefer short wavelengths of light but that they can be trained, by Pavlovian conditioning, to select specific wavelengths of light indicating that flies, like the honeybee and human, have true color vision.
In mapping the synaptic circuits of the chromatic photoreceptors R7s and R8s and their synaptic target neurons in the medulla ganglion of the peripheral visual system, we focused on amacrine neurons, which interconnect medulla columns, and medulla projection (Tm) neurons, which are thought to serve functions analogous to those of vertebrate retinal ganglion cells by processing and relaying photoreceptor information to a higher visual center. We found that the chromatic photoreceptors R7 (UV–sensing) and R8 (blue/green-sensing) provide inputs to a subset of first-order interneurons. The first-order interneurons Tm5a/b receive direct synaptic inputs from R7s while Tm9, Tm20, and Tm5c receive direct synaptic inputs from R8s. These Tm neurons also receive indirect inputs from R1–6 via L3 and relay spectral information from the medulla to the higher visual center, the lobula. In addition to the direct pathways from photoreceptors to Tm neurons, the amacrine neuron Dm8 receives input from multiple R7s and provides input for Tm5a/b/c (Figure 1).
Figure 1. Visual circuits in Drosophila
Simplified visual circuits in the Drosophila optic lobes. Color vision is mediated by four classes of transmedulla neurons (Tm5a/b/c and Tm20) downstream of the narrow-spectrum photoreceptors R7 and R8. Innate preference for ultraviolet light is mediated by a dedicated circuit composed of UV–sensing R7, the amacrine neuron Dm8, and the transmedulla neuron Tm5c. Motion detection is mediated by the lamina neurons L1–L3 and their synaptic targets, Mi1, Tm1, Tm2, and Tm3.
To relate neural connectivity to functions, we assigned components of synaptic machinery to specific connections. To directly probe the usage of neurotransmitters and receptors that determine the polarity and dynamic of signal transmission, we developed a method to profile transcripts in single neurons. We used highly specific promoter–Gal4 constructs to label single types of neurons with GFP, isolated the GFP–labeled neurons from adult fly brains, and profiled their gene expression patterns by RT–PCR. Using this method, we determined that a large percentage of the first/second-order interneurons in the chromatic circuits express the vesicular glutamate transporter, indicating that they are glutamatergic, while the remaining chromatic circuits express choline acetyltransferase and therefore are likely cholinergic. This contrasts with the motion-detection pathway, which is mostly cholinergic.
By inactivating specific neurons and examining the behavioral consequences, we previously found that the amacrine Dm8 neurons, which receive UV–sensing R7 photoreceptor inputs, are both required and sufficient for an animal's innate spectral preference to UV light. RNAi–knockdown of the vesicular glutamate transporter in the Dm8 neurons significantly reduced UV preference, suggesting that glutamatergic output of Dm8 is critical for its functions. While Dm8 provides inputs for the Tm5a/b/c transmedulla neurons, inactivating Tm5c alone abolished UV preference, indicating that Tm5c is the key downstream target for this behavior. Furthermore, RNAi–knockdown of kainate-type iGluR in Tm5c, which inhibits the neuron's ability to receive glutamatergic Dm8 inputs, significantly reduced UV preference. We developed a modified GRASP (GFP reconstitution across synaptic partners) method, which allows single-cell analysis of bona fide synaptic connections. We demonstrated that Dm8 receives 16 R7 inputs and provides inputs for 1–2 Tm5c neurons in the center of Dm8's receptive field. Thus, the R7s→Dm8→Tm5c connections constitute a hard-wired pooling circuit for detecting dim UV light.
To map the circuits that transform photoreceptor signals into color percepts, we developed a novel aversive operant conditioning assay for intensity-independent color discrimination. Single flying flies were magnetically tethered in an arena surrounded by blue/green LEDs, and the flies were then conditioned to discriminate between equiluminant blue or green stimuli. Wild-type flies can be trained in this paradigm to avoid either blue or green, while mutants lacking functional R7 and R8 photoreceptors cannot, indicating that the color entrainment requires the function of the narrow-spectrum photoreceptors R7s and/or R8s. Genetically inactivating four classes of first-order interneurons, Tm5a/b/c and Tm20, abolishes color learning. However, inactivating subsets of these neurons is insufficient to block color learning, suggesting that true color vision is mediated by several redundant pathways. The apparent redundancy in learned color discrimination sharply contrasts with innate spectral preference, which is dominated by a single pathway.
Using a similar strategy, we mapped the visual motion pathways in Drosophila. Previous studies revealed that two types of neurons, T4 and T5, signal small-field direction-selective motion responses and are downstream of the first-order neurons L1 and L2 or the ON- and OFF-channel neurons, respectively. Serial–EM reconstruction revealed that T5 receives direct synaptic inputs from four types of transmedulla neurons, Tm1, Tm2, Tm4, and Tm9. Our transcript profiling and immunohistochemistry revealed that these Tm neurons express choline acetyltransferase and therefore are likely cholinergic. Furthermore, we found that T5 expresses both nicotinic and muscarinic acetylcholine receptors. The Tm2 and Tm9 input synapses are spatially segregated on T5's dendritic arbor, thus providing candidate anatomical substrates for the two arms of elementary motion detector circuits. Based on the synaptic circuit and receptor expression profiles, we hypothesize that T5 computes small-field motion signals by integrating multiple cholinergic Tm inputs, using nicotinic and muscarinic cholinoceptors.
Dendritic development of Drosophila optic lobe neurons
Our long-term goal is to determine the molecular mechanisms guiding the assembly of neural circuits during development. Most regions of vertebrate and invertebrate brains are organized in columns and layers, which facilitate information processing and propagating. During development, axons and dendrites are routed to specific layers and columns, and spatial coincidence of axons and dendrites is a key specificity determinant for synaptic pairing. Little is known about how target neurons extend dendrites in type-specific patterns to pair with photoreceptors' pre-synaptic terminals. We are interested in how CNS neurons establish type-specific dendritic arborization patterns in three-dimensional space and how the synaptic partnership between axons and dendrites is established. Using Drosophila optic lobe neurons as a model, we aim to determine the molecular mechanisms by which CNS neurons establish appropriate sizes of dendritic trees and route dendrites to specific layers and columns to match with their presynaptic partners. Our recent study uncovered the mechanism by which photoreceptor afferents, via TGF-beta/activin, directly regulate the size of the dendritic receptive field of their target neurons.
We developed several novel techniques to analyze dendritic structures in three-dimensional space and to exploit the unique advantages of this system: (i) a dual-imaging technique that generates isotropic 3D images of dendrites by combining two confocal image stacks collected in orthogonal directions; (ii) an image-registration technique that makes use of the regular array structures of the optic lobe to standardize dendritic-branching patterns; (iii) a series of statistical methods to analyze dendritic patterns in three-dimensional space; (iv) a modified GRASP technique to detect bona fide synaptic connections at the light-microscope level.
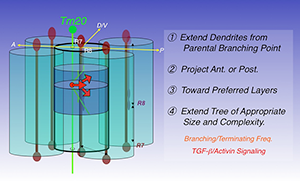
Click image to enlarge.
Figure 2. Four steps to build a dendritic tree
The transmedulla neuron Tm20 extends dendrites to specific layers in single visual columns to receive R8 photoreceptor input. Four consecutive steps are required to build a Tm20 dendritic tree: extending dendrites from branching points on axons; projecting anteriorly; projecting toward preferred medulla layers; extending trees of appropriate sizes and complexity. In the last step, R8 photoreceptors provide TGF-beta/activin, acting at short-range, to regulate branching and the termination frequency of Tm20 dendrites.
Using these techniques, we analyzed the wild-type dendritic morphologies of three medulla neuronal types, Tm2, Tm9, and Tm20. Tm20 neurons receive input from R8 photoreceptors in their cognate columns and mediate color vision, while Tm2 neurons receive inputs from the achromatic R1-6 via L2 and L4 in their cognate columns to mediate motion detection. Morphometric analyses revealed that (i) the patterns are stereotypic but the detailed branching pattern and topology are not conserved; (ii) the synaptic partnerships are robust and specific; (iii) the layer-specific routing and polarized extension of dendrites are the two most critical determinants of type-specific dendritic patterns; (iv) these Tm neurons elaborate dendrites to single medulla columns, consistent with their functions in processing retinotopic information. In contrast, the Dm8 amacrine neurons form a wide dendritic field to cover approximately 16 medulla columns to receive R7 photoreceptor inputs. Based on the results, we hypothesize that dendritic development in the optic lobe neurons proceeds in four distinct processes: (i) initiating dendritic extension from axons in specific layers; (ii) projecting dendrites in a specific planar direction (anteriorly or posteriorly); (iii) routing dendrites toward specific medulla layers; (iv) elaborating a dendritic tree of an appropriate size (Figure 2). The processes are likely mediated by distinct molecular mechanisms.
To determine the molecular mechanisms guiding dendritic development, we screened various mutants for their functions in Tm20 and Dm8 dendritic development. We found that mutations in the components of the TGF-beta/activin signaling pathway affect the size of the neurons' dendritic fields. R7- and R8-photoreceptor–derived activin selectively restricts the dendritic fields of the photoreceptors' respective postsynaptic partners, Dm8 and Tm20, to the size appropriate for their functions; Tm20's dendrites occupy a single column while Dm8's dendrites cover about 16 columns. Canonical activin signaling in Tm20 promotes dendritic termination without affecting dendritic routing direction or layer. Mutant Tm20 neurons lacking components of the activin signaling pathway over-expanded their dendritic fields and formed incorrect synapses with neighboring photoreceptors, thereby losing the one-to-one correspondence with R8s. Similarly, mutant Dm8 neurons devoid of activin signaling over-expanded their dendritic field, while mutant Dm8 neurons with overactive activin signaling had smaller dendritic fields. Our finding that photoreceptor-derived activin controls the dendritic development of their synaptic targets suggests that anterograde signaling is an effective mechanism for coordinating afferent target development late in development. The use of afferent-derived activin to regulate dendritic patterning provides an adaptable and self-compensating mechanism for afferents to control the receptive-field sizes of their synaptic partners.
Publications
- Ting C-Y, McQueen PG, Pandya N, Lin T-Y, Yang M, Reddy OV, O’Connor MB, McAuliffe M, Lee C-H. Photoreceptor-derived activin promotes dendritic termination and restricts the receptive fields of first-order interneurons in Drosophila. Neuron 2014;81:830-846.
- Karuppudurai T, Lin T-Y, Ting C-Y, Pursley R, Melnattur KV, Diao F, White BH, Macpherson LJ, Gallio M, Pohida T, Lee C-H. A hard-wired glutamatergic circuit pools and relays UV signals to mediate spectral preference in Drosophila. Neuron 81;603-615.
- Melnattur KV, Pursley R, Lin T-Y, Ting C-Y, Smith PD, Pohida T, Lee C-H. Multiple redundant medulla projection neurons mediate color vision in Drosophila. J Neurogenet 2014;E-pub ahead of print.
- Shinomiya K, Karuppudurai T, Lin T-Y, Lu Z, Lee C-H, Meinertzhagen IA. Candidate neural substrates for off-edge motion detection in Drosophila. Curr Biol 2014;1062-1070.
- Kundu M, Kuzin A, Lin T-Y, Lee C-H, Brody T, Odenwald WF. cis-Regulatory complexity within a large non-coding region in the Drosophila genome. PLoS One 2013;8:e60137.
Collaborators
- Mikko Juusola, PhD, The University of Sheffield, Sheffield, United Kingdom
- Matthew McAuliffe, PhD, Division of Biomedical Imaging Research Services Section, CIT, NIH, Bethesda, MD
- Philip McQueen, PhD, Mathematical and Statistical Computing Laboratory, CIT, NIH, Bethesda, MD
- Ian Meinertzhagen, PhD, DSc, Dalhousie University, Halifax, Canada
- Ward F. Odenwald, PhD, Laboratory of Neurochemistry, NINDS, Bethesda, MD
- Nishith Pandya, BA, Division of Biomedical Imaging Research Services Section, CIT, NIH, Bethesda, MD
- Thomas Pohida, MSEE, Division of Computational Bioscience, CIT, NIH, Bethesda, MD
- Randy Pursley, MSEE, Division of Computational Bioscience, CIT, NIH, Bethesda, MD
- Mark Stopfer, PhD, Program in Developmental Neuroscience, NICHD, Bethesda, MD
- Benjamin White, PhD, Laboratory of Molecular Biology, NIMH, Bethesda, MD