You are here: Home > Section on Neural Developmental Dynamics
Building the Zebrafish Lateral Line System
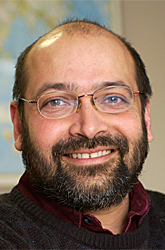
- Ajay Chitnis, MBBS, PhD, Head, Section on Neural Developmental Dynamics
- Gregory Palardy, BS, Research Technician
- Chongmin Wang, MS, Research Technician
- Damian E. Dalle Nogare, PhD, Research Fellow
- Harsha Mahabaleshwar, PhD, Postdoctoral Fellow
- Uma Neelathi, PhD, Postdoctoral Fellow
- Jeffery Head, BS, Postbaccalaureate Intramural Research Training Award Fellow
- Caitlin Fox, BS, Graduate Student
Our goal is to determine how the architecture of the mature nervous system emerges as a consequence of local interactions between cells during early development. We use a combination of cellular, molecular, genetic, and computational tools to understand how cells differentiate in distinct patterns in the various compartments of the zebrafish nervous system. We analyze zebrafish mutants and embryos microinjected with morpholinos or mRNA to alter gene function. Our current studies examine how the posterior lateral line (PLL) system is built in the zebrafish nervous system. Our goal is to define gene-regulatory networks that coordinate cell fate, morphogenesis, and migration in the lateral line system and, based on these studies, to build computational models that help us understand how this relatively simple and extremely accessible sensory system in zebrafish builds itself.
The lateral line is a mechanosensory system that detects water flow and consists of sensory organs called neuromasts, which are distributed in a stereotypical pattern over the surface of the zebrafish. Each neuromast has sensory hair cells at its center. The hair cells are surrounded by support cells, which serve as progenitors for the production of more hair cells during growth and regeneration of neuromasts. The development of this superficial sensory system in zebrafish can be observed easily in live embryos, with transgenic lines expressing fluorescent proteins in specific subsets of cells of the lateral line system. In addition, a range of genetic and cellular manipulations can be used to investigate gene function.
The function of sensory hair cells in fish neuromasts is remarkably similar to that of hair cells in the vertebrate ear. Furthermore, the gene-regulatory network that specifies neuromast hair cells is very similar to that specifying hair-cell fate in our ear. Like hair cells in our ears, neuromast hair cells can be damaged by exposure to drugs such as aminoglycosides and to copper ions and noise. However, unlike our ears, in which loss of hair cells can be permanent, neuromast hair cells have the remarkable ability to regenerate. Hence, the lateral line system serves as an excellent model system with which to understand development and for developing strategies to engineer regeneration of sensory hair cells.
The PLL system is initially established by the PLL primordium (PLLp), a cluster of about a 100 cells that migrate from the ear to the tip of the tail, periodically depositing neuromasts. Recent studies showed that the mechanisms determining and guiding collective migration and deposition of cells from the PLLp are remarkably similar to those determining the collective migration of metastatic cancer cells. Hence, the lateral line system has also recently emerged as an excellent system for studying the biology of metastatic cancer cells.
Our expectation is that understanding the gene-regulatory network that coordinates cell fate and morphogenesis of the zebrafish lateral line system will ultimately have a profound impact on translational studies that address a wide range of issues, including the development and regeneration of sensory systems and therapies directed at limiting the spread of cancer through metastasis.
Modeling signaling and migration of the PLLp with agent-based computational models
Cells communicate with each other to determine the self-organization of cell communities within developing organs. Understanding the role that individual signaling pathways play in these interactions has helped define simple rules by which cells interact with their environment and their neighbors to influence cell behavior, proliferation, and differentiation. Agent-based computational models allow us to visualize how characteristic behaviors and patterns of differentiation emerge when large numbers of cells interact following simple rules that we can define based on genetic, molecular, and cell-biological analysis of cell-cell interactions in the embryo. We have been examining collective migration of cells in the PLLp with time-lapse imaging in live zebrafish embryos, and we built agent-based models of this system based on what we learned from our observations. The computational models allow us to determine whether our current understanding is adequate to account for various behaviors of the cells in the PLLp. While recapitulation of complex emergent behaviors in computer simulations provides support to our current hypotheses, failure of the computational models to reproduce specific behaviors helps identify missing pieces in our understanding.
As stated above, formation of the PLL is spearheaded by the PLLp, the cells of which migrate from the ear to the tip of the tail, periodically depositing sensory organs called neuromasts from the trailing end. During its journey, the PLLp follows a path defined by the expression of the chemokine Cxcl12a (also called Sdf1a) by cells of the horizontal myoseptum. While the differential expression of the chemokine typically determines chemotactic migration of a variety of cells, the unidirectional migration of the PLLp along the horizontal myoseptum is not determined by the inherent differences in Cxcl12a expression along its path. Instead, it is the polarized expression of two distinct chemokine receptors, Cxcr4b in leading cells and Cxcr7b in trailing cells, that allows the PLLp to migrate effectively along a relatively uniform stripe of chemokine expression. While leading Cxcr4b receptor–expressing cells are capable of responding to the Cxcl12a ligand with protrusive activity, trailing Cxcr7b receptor–expressing cells are not. However, interaction of Cxcl12 with both receptors is followed by internalization of the bound ligand–receptor complex from the cell’s surface, which is expected to deplete Cxcl12a from the immediate environment of the PLLp. This ensures that leading Cxcr4b cells always encounter the higher levels of Cxcl12a ahead of the migrating PLLp, in which direction leading cells continue to move. On the other hand, expression of the non-responsive Cxcr7b receptor at the trailing end ensures that the PLLp does not move in the opposite direction.
We constructed an agent-based model using the Netlogo modeling environment to visualize how the functional differences in the chemokine receptors, coupled with local depletion of Cxcl12a, contribute to polarized migration of the PLLp. The programming environment consists of individual, mobile agents called turtles, where multiple breeds of turtles can be defined that behave according to distinct sets of defined rules. In addition there are links that form visco-elastic connections between defined sets of turtles and individual non-motile agents called patches, which define the environment in which the motile agents operate. In our models, patches are used to represent the substrate upon which the PLLp migrates and to define the source of Cxcl12a. The PLLp is made of two breeds of turtles: a leading compartment of Cxcr4-turtles and a trailing compartment of Cxcr7b-turtles. Links connect adjacent turtles and model adhesive interactions between cells of the PLLp. Movement of the PLLp is determined by the movement of Cxcr4b–expressing turtles at the edge of the PLLp. This indirectly determines movement of trailing cells by virtue of the visco-elastic links connecting neighboring turtles. The model recapitulates polarized migration of the PLLp and allows us to explore how change in the relative size of Cxcr4b and Cxcr7b domains affects PLLp migratory behavior.
We cut the migrating PLLp with a laser so as to release a leading un-polarized fragment with only Cxcr4b–expressing cells and a remaining still polarized trailing fragment with both Cxcr4b and Cxcr7b cells in the two fragments, which produced distinct and characteristic behaviors in the resulting two fragments. To challenge our computational model, we sought to determine whether it could recapitulate these behaviors following a simulated cut of the PLLp. Our simulations were able to recapitulate bilateral stretching behavior of the leading fragment. However, they could not recapitulate the polarized protrusions of the trailing fragment toward the remaining leading fragment. This suggested that some behaviors of the PLLp cells could not be accounted for by assumptions of our chemokine signaling–based model but might be related to migratory behavior determined by other signaling pathways. The possibility that a mechanism independent of chemokine signaling was responsible for trailing cell behavior in this context was confirmed by the observation that, when the laser-cut experiment was repeated in the embryo in the presence of a chemokine signaling inhibitor, the polarized protrusions of the trailing toward the leading fragment persisted, even though bilateral stretching of the leading fragment was lost.
The failure of our computational model prompted us to investigate the potential role of FGF (fibroblast growth factor) signaling in determining behavior of the trailing cells toward the leading cells. Our experiments eventually demonstrated that leading cells are the source of FGFs that provide a chemotactic cue for trailing cells; they play "follow-the-leader" leader as they follow leading cells that secrete FGFs.
The lessons learned from the extremely accessible and easy-to-image PLLp system will eventually contribute to our understanding of much less accessible and difficult-to-study metastatic cancer cells, whose collective migration appears to be directed by principles and signaling mechanisms that have striking parallels with those we are investigating in the zebrafish lateral line system.
Publications
- York AG, Chandris P, Nogare DD, Head J, Wawrzusin P, Fischer RS, Chitnis A, Shroff H. Instant super-resolution imaging in live cells and embryos via analog image processing. Nat Methods 2013;10:1122-1126.
- Matsuda M, Nogare DD, Somers K, Martin K, Wang C, Chitnis AB. Lef1 regulates Dusp6 to influence neuromast formation and spacing in the zebrafish posterior lateral line primordium. Development 2013;140:2387-2397.
- Dalle Nogare D, Somers K, Rao S, Matsuda M, Reichman-Fried M, Raz E, Chitnis AB. Leading and trailing cells cooperate in collective migration of the zebrafish posterior lateral line primordium. Development 2014;141(16):3188-3196.
Collaborators
- Hari Shroff, PhD, Laboratory of Molecular Imaging and Nanomedicine, NIBIB, Bethesda, MD
Contact
For more information, email chitnisa@mail.nih.gov or visit neuroscience.nih.gov/Faculty/Profile/ajay-chitnis.aspx.