You are here: Home > Section on Molecular Regulation
Global Regulation of Gene Expression by ppGpp
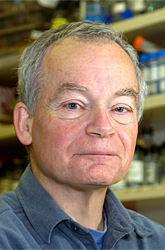
- Michael Cashel, MD, PhD, Head, Section on Molecular Regulation
- Katarzyna Potrykus, PhD, Research Fellow
- Rajendran Harinarayanan, PhD, Research Scholar
The long-term focus of our laboratory has been on uncovering the molecular-biological roles of two naturally occurring nucleotides, commonly abbreviated as (p)ppGpp. These are analogs of GTP and GDP with ribosyl 3' pyrophosphate residues; the compounds function as important second messengers in bacteria and plants. In this report, we abbreviate both compounds as ppGpp. In bacteria and plants, generalized nutritional stress results in an increase in cellular levels of ppGpp. Examples of effective nutritional stress for ppGpp regulation include starvation for sources of nitrogen, carbon, amino acids, phosphate, iron, oxygen, or specific vitamins (1). When ppGpp levels rise in response to either stress or a result of stress-free artificial genetic manipulation, similar complex global regulatory effects on gene expression ensue at the transcriptional level. In Escherichia coli, regulatory effects are gene-specific, but with both inhibitory and stimulatory components; overall, the transcriptional activities of about one-third of the genomic repertoire are modulated. Extensive physiological modulation occurs through interconnecting regulatory circuits (4). The details of these global mechanisms at the level of transcription continue to hold our central interest, and we use molecular microbiology as the most genetically tractable approach (2, 3).
The search for ppGpp in animal cells
Recently, two reports provided strong circumstantial evidence that ppGpp and its regulatory effects on gene expression might be biologically ubiquitous rather than limited to bacteria and plants, as commonly thought. In one report, genes encoding ppGpp–specific hydrolases, called Mesh1, were found in worms, flies, and humans (Sun et al., Nat Struct Biol 2010;17:1188). In the other report, bioinformatic analysis of genomes identified the hydrolases in animals as well as in archaeal species (Atkinson et al., PLoS One 2011;6(8):e23479). The animal ppGpp–hydrolytic enzymes are close structural and catalytic homologs of the bacterial enzymes, and the animal genes function interchangeably with their bacterial counterparts when expressed in bacterial mutants. Conversely, when expressed in animal cells, bacterial enzymes appear to function interchangeably with animal enzymes. When excess ppGpp is produced in flies by over-expression of an E. coli synthetase, embryogenesis is perturbed with a phenotype similar to that seen with a deletion of the Mesh1 ppGpp hydrolase gene. This is consistent with the notion that the role of the animal hydrolase in development involves regulation of ppGpp itself. In addition, transcriptional profiles of global regulatory effects of excess ppGpp in flies are reminiscent of similar effects in bacteria. Finally, strong empirical correlations suggest that finding a ppGpp–specific hydrolase in animal cells is prima facie evidence that the ppGpp nucleotide is present and formed by a ppGpp hydrolase. Available bacterial and plant genomes reveal that a ppGpp–specific hydrolase is invariably present together with ppGpp synthetase; conversely, genomes are never found without one or the other enzyme alone; instead, both are always missing. However strong the correlation, it is not proof that ppGpp exists in animal cells.
Our goal is to obtain a conclusive answer to the question of whether ppGpp has regulatory effects on gene expression in animals. Direct identification of the ppGpp nucleotide in animal cells is a formidable task despite the high sensitivity of chemical techniques such as mass spectrometry. The reason is that the extreme metabolic lability of ppGpp (a half life in bacteria of about 20 sec) is coupled with its chemical instability under conditions normally used to block its metabolic degradation (acid and alkali). Instead, we adopted the classical genetic approach of screening for ppGpp synthetase genes with cDNA libraries expressed in bacteria. The libraries are constructed from Drosophila embryonic stages that express the Mesh1 hydrolase gene as judged by PCR assays. We are using a variety of growth tests capable of detecting a picomolar to micromolar range of bacterial ppGpp levels. The tests successfully detected plant ppGpp synthetases. A collaboration with James Kennison and Monica Cooper provided us with access to mRNA from embryo, larva, and pupa developmental stages. As a control, we verified the presence of Mesh1 mRNA at all three stages by PCR. We are in the process of transforming bacterial mutants with these libraries and screening colonies for the presence of ppGpp synthetase.
Alternatively, it can be imagined that the Mesh1 hydrolase could degrade a toxic substance other than ppGpp. Presumably, this alternative hydrolase substrate would share structural similarities with ppGpp. Available evidence stipulates that this substrate, like ppGpp, is also synthesized by overexpression of E. coli ppGpp synthetase in animal cells. This appears to be a severe constraint, given that the E. coli synthetase is known to have a receptor substrate specificity limited to pyrophophosphoryl transfer to only 5′ di- or triphospho-ribonucleotides with only 6-hydroxy purines as the nucleobase (inosine or guanosine derivatives). We will undertake structure-specificity studies of hydrolysis of various pyrophosphorylated substrates with purified Mesh1 proteins to explore the nature of candidate substrates.
A genetic survey to identify effects in the absence of ppGpp of imbalances of transcription factors GreA, GreB, and DksA in E. coli
A major goal of our laboratory is to define roles for ppGpp that integrate bacterial stress responses through gene-specific positive and negative regulatory effects on transcription (4). It is known that ppGpp can directly affect specificity of transcription at the promoter level, but without participation of DNA sequence recognition proteins other than RNA polymerase (RNAP). Instead, a protein called DksA occupies the secondary channel of RNAP, which together with ppGpp, alters transcription specificity. It is important that most of these known transcription effects at physiological levels are exerted by the combination of DksA and ppGpp, not by either factor alone. Several other proteins share the same structural features of DksA, such as GreA and GreB. The GreA/B proteins are better known as transcription factors that function during elongation to relieve paused or arrested transcription rather than initiation. Despite structural similarities, DksA apparently does not possess GreA/B functions, and GreA/B will not substitute for DksA to regulate transcription initiation with ppGpp in the same manner as DksA. Nevertheless, preliminary indications suggest that the functions of GreA/B interface with DksA, particularly in the absence of ppGpp.
Earlier work led us to appreciate that, in some respects, the activities of Gre factors are more complex than predicted from their assigned roles in reversing transcriptional pausing or arrest. We have chosen to use a genetic approach to discover the breadth of unexpected interactions between these transcription factors. We screen for phenotypic effects of overexpressed GreA, GreB, or DksA in a wild-type hosts and in various combinations of hosts deleted for greA, greB, and/or dksA. The phenotypes affect the nine amino-acid requirements of ppGpp-deficient (ppGpp0) cells as well as a related subset of amino-acid requirements of dksA mutants. The analyses reveal that DksA and GreA/B may show similar and even redundant functions, despite the intrinsic differences mentioned above. One example is that GreA overproduction can satisfy the amino-acid requirements of a dksA mutant, and another is that GreA or DksA overexpression can partially reverse some of the same amino-acid requirements of ppGpp0 cells. Using microarray transcription profiles, we verified conclusions about shared functions for many genes. Intriguingly, we also find instances in which GreA and DksA act in opposition. An example of opposite effects at the phenotypic level is complete reversal of the amino-acid requirements of ppGpp0 cells by overexpression of GreA only in the absence of DksA and vice versa. As judged by transcription profiling, more genes—331—are affected by GreA overproduction when DksA is absent than when DksA is present—45. Profiling also provides evidence that the strongest activation by GreA occurs for gadA and gadE genes and is verified through using PgadA–lacZ and PgadE–lacZ promoter transcriptional fusions as reporters. The construction of these fusions also localizes activation to the level of transcription initiation rather than to the level of elongation. We also studied mutants of GreA and DksA that abolish their standard elongation or regulation activities with the promoter–lacZ fusions. The failure of these mutants to substantially alter regulation leads us to conclude that the newly identified regulatory features are novel and not due to the usual activities of these secondary channel proteins. This reinforces our earlier notions about an interesting and complex interplay among GreA, GreB, and DksA in the absence of ppGpp, which could involve promoter activation and competition for RNA polymerase at the level of binding, secondary channel occupancy, or both, not to mention mutual control of one another's gene expression. The work localizes some of these interactions for specific genes and regulators, whose further study should lead to the discovery of new regulatory mechanisms at the molecular level.
Overall, our current work documents a much more complex and generalized regulatory interplay among GreA, GreB, and DksA than previously thought. The following features are found for this regulatory phenomenon: (i) GreA plays a major and GreB a minor role in the ppGpp-DksA regulatory network; (ii) regulation is particularly evident when ppGpp is absent; (iii) regulation controls growth through activation of otherwise impaired amino-biosynthetic pathways; (iv) regulation is novel, given that it persists with mutants of key residues of GreA and/or DksA; and (v) examples of the regulatory interplay acting on RNA polymerase can vary widely from redundant to opposing functions (5).
Publications
- Szalewska-Palasz A, Potrykus K, Cashel M, Wegrzyn G. Starvation of bacteria for amino acids as an example of prokaryotic response to nutritional deprivation. In: Merkin TE, ed. The Biology of Starvation in Humans and Other Organisms 2011;e-Book.
- James T, Cashel M, Hinton D. A mutation within the beta subunit of Escherichia coli RNA polymerase impairs transcription from bacteriophage T4 middle promoters. J Bacteriol 2010;192:5580-5587.
- Potrykus K, Murphy M, Philippe N, Cashel M. ppGpp is the major source of growth rate control in E. coli. Environ Microbiol 2010;13:563-576.
- Edwards AN, Paterson-Fortin LM, Vakulskas CA, Mercante JW, Potrykus K, Vinella D, Comancho MI, Fields JA, Thompson SA, Georgellis D, Cashel M, Babitzke P, Romeo, T. Circuitry linking the Csr and stringent response global regulatory systems. Mol Microbiol 2011;80:1561-1580.
- Vinella D, Potrykus K, Murphy H, Cashel M. Effects on growth by changes of the balance between GreA, GreB and DksA suggest mutual compeitition and functional redundancy in Escherichia coli. J Bacteriol 2011;[Epub ahead of print].
Collaborators
- James Kennison, PhD, Program in Genomics of Differentiation, NICHD, Bethesda, MD
- Monica Cooper, BA, Program in Genomics of Differentiation, NICHD, Bethesda, MD
Contact
For more information, email cashel@mail.nih.gov or visit smr.nichd.nih.gov.