Translational Biophotonics in Developmental Disorders and Diseases
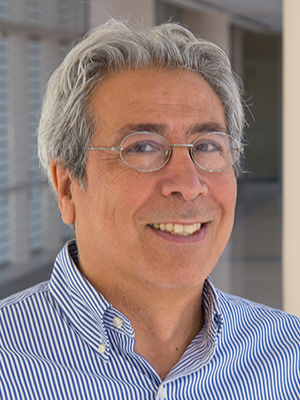
- Amir H. Gandjbakhche, PhD, Head, Section on Translational Biophotonics
- Hadis Dashtestani, PhD, Postdoctoral Fellow
- Thien Nguyen, PhD, Postdoctoral Fellow
- Jinho Park, PhD, Postdoctoral Fellow
- Soongho Park, PhD, Postdoctoral Fellow
- Wan-Chun Su, PhD, Postdoctoral Fellow
- Wei Lun Huang, MS, Intramural Research Training Award Student
- Emily Blick, BS, Postbaccalaureate Fellow
- Aaron Buckley, BS, Postbaccalaureate Fellow
- Sara Johnson, BS, Postbaccalaureate Fellow
- William Martin, BS, Postbaccalaureate Fellow
- John Millerhagen, BS, Postbaccalaureate Fellow
- Vinay Veluvolu, BS, Postbaccalaureate Fellow
- Marc Bornstein, PhD, Special Volunteer
- Han-Shin Hahn, PhD, Special Volunteer
- Kosar Khaksari, PhD, Special Volunteer
- Siamak Aram, PhD, Guest Researcher
Brain imaging and spectroscopy of developmental disorders
We used the unimodal and multimodal approaches of functional near-infrared spectroscopy (fNIRS) and electroencephalogram (EEG) to conduct two distinct lines of research: (1) to investigate the developmental trajectories of neural activity associated with social-cognitive and motor signs in children with/without elevated risk for developmental disorders; and (2) to validate the fNIRS findings with that of the gold standards of EEG and/or functional magnetic resonance imaging (fMRI) during social cognitive tasks. Our goals are to validate the use of the child-friendly neural imaging tool fNIRS in early detection of developmental disorders and for predicting later developmental trajectories. To do so, we continued our collaborations with Audrey Thurm and Nathan Fox to study the development of the mirror neuron network (MNN) in infants. The MNN is associated with the development of sophisticated social-cognitive behaviors, which emerge during infancy (e.g., complex imitation, decoding emotional states). Modeling MNN development will create a sensitive measure of deviations in social-cognitive development before clinical behavioral deficits can be detected. MNN activation has already been associated with mu desynchronization (i.e., attenuation of EEG power in the alpha frequency), using EEG. Our research group extended these findings to fNIRS–related hemodynamic changes of the MNN. Moreover, we combined the use of EEG (which has high temporal resolution) with fNIRS (which provides a more precise spatial resolution of neural activity) to provide new insights into the MMN findings.
So far, five manuscripts were published from this work [Condy EE et al. Front Hum Neurosci 2021;15:627983; Miguel HO et al. PLoS One 2021;16:e0253788; Nguyen et al. Brain Sci 2021;11:397; and References 1 & 2], and one manuscript is under review. First of all, we conducted a thorough review of the literature summarizing previous neural findings in the MNN using fNIRS in adults. We then conducted our own fNIRS study to examine the hemodynamic changes in the MNN during a live action-observation and execution task. Thirty adults participated in our study in which they either reached for or observed an experimenter reach for objects. Their hemodynamic changes were recorded by fNIRS probes placed on the sensorimotor and parietal regions (bilateral superior parietal lobule [SPL] and bilateral inferior lobule). The inferior parietal lobule (IPL), right supramarginal gyrus (SMG), and right angular gyrus (AG) were activated in both observation and execution conditions. Moreover, during execution, greater activation was found in the left precentral and postcentral regions than during observation. Besides the level of hemodynamic changes, previous neuro-imaging research suggested lateralized brain activity in the MNN during action execution, with greater activation in the contralateral than in the ipsilateral hemisphere. However, it is unknown whether the same lateralization could be found during action observation. We therefore used fNIRS to investigate the lateralization of cortical activation during a similar live action-observation and action-execution task. Our results suggested brain lateralization during action execution but not observation. In addition, we found that hand preference and dexterity between the right and left hands are related to brain lateralization patterns [Reference 1]. Our results are consistent with previous fMRI findings, supporting fNIRS as an applicable and valid method for studying the neural mechanisms of cognitive and motor signs. We also investigated the neural connectivity over MMN and its correlations with autistic traits in neurotypical adults. We defined the MNN connections with the regions that showed significantly greater connectivity in both action-execution and action-observation tasks than in a baseline, resting-state. Correlations between connectivity and autistic traits were also investigated using Pearson correlations. Strong MNN connections were found over the right precentral, right SMG, left IPL, left postcentral, and between left SMG–left AG. In addition, individuals with higher subclinical autistic traits present higher connectivity in both action execution and action-observation conditions. Positive correlations between MNN connectivity and subclinical autistic traits can be used in future studies to investigate the MNN in a developing population with ASD.
Besides the unimodal studies, we used a multimodal approach, i.e., simultaneous recordings of fNIRS and EEG, to reveal new insights in neural activity during action execution, observation, and imagery [Reference 2]. We simultaneously recorded fNIRS and EEG–based neural activity during the live action observation-execution and imagery tasks. Benefitting from concurrent recordings of brain hemodynamic and electrophysiological responses, we used a novel method, structured sparse multiset Canonical Correlation Analysis (ssmCCA), to find linear transforms of each modality and maximize the correlations between the projections of each modality. Our findings from 21 right-handed participants confirmed neural activity over the MNN system (e.g., central and parietal regions) during all three conditions. Unimodal analyses revealed differentiated activation between conditions; however, the activated regions did not fully overlap across the two modalities (fNIRS: left pre- and post-central, right angular gyrus; EEG: bilateral central, right frontal, and parietal). The discrepancies might be because fNIRS and EEG detect different signals. Using fused fNIRS–EEG data, we consistently found activation over the left inferior parietal lobe, superior marginal gyrus, and post-central gyrus during all three conditions, suggesting that our multimodal approach identifies a shared neural region associated with MNN (Figure 1). The study highlights the strengths of the multimodal fNIRS–EEG fusion technique for studying the MNN. Neural researchers should consider using the multimodal approach to validate their findings. In the future, we will use the multimodal approach to conduct longitudinal studies in children with or without elevated risks for developmental disorders. We believe that the study will facilitate early detection and intervention for children with developmental disorders.
Figure 1. Simultaneous recording of fNIRS and EEG
(A, right) Color-coded covariation in fNIRS and EEG recordings.
(B, below) Regions, their cross-modality correlations, and the corresponding p-values.
Execution | Observation | Imagination | |||||||
---|---|---|---|---|---|---|---|---|---|
Correlation | Corresponding Region | P-Value | Correlation | Corresponding Region | P-Value | Correlation | Corresponding Region | P-Value | |
Comp 1 | 0.3905 | Left IPL | 0.055 | 0.4823 | Left IPL | 0.0457 | 0.4418 | Left SMG | 0.0573 |
Comp 2 | 0.3821 | Left POCG | 0.0581 | 0.3753 | Left SMG | 0.0498 | 0.4080 | Left POCG | 0.0585 |
Comp 3 | 0.3548 | Left SMG | 0.0624 | 0.3310 | Left POCG | 0.053 | 0.3017 | Left IPL | 0.0638 |
Comp 4 | 0.3350 | Left PRCG | 0.0653 | 0.3351 | Left PRCG | 0.0617 | 0.300 | Left PRCG | 0.0664 |
COVID-19 point-of-care biosensor
As a result of the recent outbreak and spread of various mutants of coronavirus disease 19 (COVID-19), many people are experiencing high fever, acute respiratory diseases, and, in severe cases, death. In order to prevent the spread of COVID-19, a monitoring system that can monitor the status of the infected person real-time while minimizing contact with the infected person is needed. Patients with infectious viral diseases such as COVID-19 experience hypoxia, hypoxemia, and lung dysfunction caused by the destruction of lung cells by the virus, which changes the tissue oxygen saturation and body temperature of the infected patient. The purpose of our study is to develop a multimodal sensor device for measuring changes in acute respiratory disease–infected patients, and to develop a classification technology using data obtained from a complex sensor device. Such devices could become important tools for monitoring disease onset, progression, and recovery in the future.
At the beginning of the COVID-19 pandemic, we conducted a review study on the efficacy of infrared thermography for screening infectious diseases and found that the technology was not reliable. We therefore proposed using multiple vital signs such as blood and tissue oxygen saturation levels, to screen for an infectious disease. To prove the utility of our proposal, we started a multisite study at NIH and the University of British Columbia in Vancouver, Canada. In collaboration with Babak Shadgan, data were collected on 24 healthy volunteers during normal breathing, restricted breathing, and rapid breathing through a clinical protocol approved by the University of British Columbia. Tissue oxygenation levels and hemodynamics responses, including oxy-, deoxy-, and total hemoglobin, were measured using an NIRS device. The device consists of a multi-wavelength LED emitting light at 760 nm and 850 nm, and three photodiode detectors 3, 3.5, and 4 cm away from the LED. The NIRS device was placed over the participant's chest during data acquisition. In order to obtain signals similar to the breathing patterns experienced by COVID-19–infected patients, three breathing patterns, i.e., normal breathing, restricted breathing, and rapid breathing, were defined. During normal breathing, participants were instructed to breathe in a comfortable state. During the restricted breathing, nasal breathing was restricted by blocking the participant's nose with a clip, while mouth breathing was done through a breathing trainer. During the rapid breathing, participants were instructed to take about 25 shallow and rapid breaths per minute. A classification algorithm was based on machine learning for breathing patterns. We evaluated whether meaningful features can be detected from data acquired using the NIRS device and to classify various breathing patterns using machine learning technology (Figure 2). The maximum/minimum peaks of the O2Hb data signal were detected. The interval and amplitude differences between the detected peaks were then used to generate two features: respiratory interval and breathing depth. The generated features and mean O2Hb were used for learning and classification using random forest machine learning technology. We achieved an accuracy of 86% [Reference 3]. We are exploring the use of Deep Learning methods for real time classification.
Figure 2. Classification of loaded, rapid, and normal breathing for monitoring COVID-19 infection
Machine learning techniques permit the classification of different breathing patterns for assessing respiratory diseases such as COVID-19.
Placenta oxygenation: from basics to point of care
The placenta plays an essential role in the health of both mother and fetus. An abnormal placenta is associated with pregnancy complications such as preeclampsia, fetal growth restriction, fetal death, preterm labor, and other complications. NIRS is an optical method for non-invasive measurement of blood oxygenated and deoxygenated hemoglobin and tissue oxygenation in deep tissue layers such as brain, muscle, and placenta. However, studies examining placental oxygenation levels have yielded conflicting results, discrepancies that may be the result of unknown placental scattering coefficients used for oxygenation calculations or differences in patient populations. A major problem in the assessment of placental oxygenation using NIRS arises from the anatomical location of the organ; taking that into account (e.g., skin, adipose tissue, uterine wall), we designed a new wearable depth-resolving NIRS device featuring six source-detector distances in the range of 10–60 mm. Distinct source and detector distances scan different tissue depths to help distinguish between placental and maternal oxygenation. The device also uses two light sources with wavelengths of 760 nm and 840 nm so that it is sensitive to changes in blood oxyhemoglobin and deoxyhemoglobin. We evaluated the performance of the NIRS device by observing changes in the optical properties of a placental-mimicking phantom at a depth of 25 mm. In addition to evaluating the accuracy and validating the performance of the custom NIRS device, we performed in vivo oxygen measurements on two human subjects using a wearable NIRS device in various parts of the body, including arms, calves, and abdomen. We also compared the wearable NIRS device with a time-domain NIRS system (TRS-41 system, Hamamatsu Photonics, Japan) on different parts of the body, including the arms, calves, and abdomen, to evaluate the accuracy and validate performance. We found an average error of 2.7% ± 1.8% between the two devices/systems, an agreement between the measurements of the wearable NIRS device and the well established TRS system that validates the high accuracy of the device in in vivo tissue oxygenation measurements.
We then used the NIRS device to measure placental oxygenation in vivo in 12 volunteers from the Advanced Obstetrics and Gynecology Research Center located at Detroit Medical Center (DMC). Measurements were performed at three locations: upper, middle, and lower parts of the placenta. Of the 12 subjects, five had maternal pregnancy complications such as short cervix, hypertension, or polyhydramnios. Eleven of the 12 participants delivered at the DMC. After delivery, the placentas of 10 of 11 participants were transferred to the DMC pathology department to examine the lesion. Chronic or acute lesions were found in five placentas, four of which were from participants with maternal pregnancy complications. The level of placental oxygenation was calculated using the intensity of backscattered light at the appropriate light source-detector separation. For each patient, three oxygenation levels at the three measurement sites were used: the upper, middle, and lower parts of the placenta. Our results suggest a possible relationship between placental oxygenation levels and pregnancy complications and placental pathology. However, the sample size used in this study is small (12 participants). We will conduct further studies to include more subjects. Results from this study have been published [Reference 4]. We are performing Monte Carlo simulations on the four-layer model to better understand the experimental results and to create theoretical indicators. Simulations are based on thickness and scattering and absorption coefficients of all maternal layers (dermis, adipose, uterus/rectus) and placenta. Based on Monte Carlo's random-walk theory and experimental results, we are attempting to develop a system that can analyze the characteristics of light scattering and propagation in the mother's maternal layers and more clearly measure the oxygenation levels of the mother's placenta. We are currently waiting for the results of the cord blood gas analysis at delivery, pregnancy outcomes, and placental pathology of 24 participants in DMC, whom we have measured. In the meantime, a two-layer model, based on Monte Carlo simulations and tissue thicknesses, is being developed to calculate the oxygenation level of the second layer. We consider maternal skin, adipose, and uterus as the first layer and the placenta as the second layer. The calculated placental oxygenation will be correlated with the oxygen gas content in the cord blood and pregnancy outcomes as well as placental pathology (Figure 3).
Figure 3. Wireless, wearable biosensors for placental oxygenation
(A) A wireless, flexible, and wearable multimodal biosensor, which consists of two multiwavelength LEDs, four PDs, and an accelerator, to monitor pregnancy health.
(B) During a measurement the biosensor was being placed on the abdomen, above the placenta, of a pregnant woman.
As a result of the COVID-19 pandemic, patient recruitment at the Center for Advanced Obstetrical Care and Research of the Perinatology Research Branch was interrupted. Although we were able to perform measurements on 24 pregnant women during our last visit to DMC in September, it will be difficult to recruit a larger population in future. Hence, we initiated a collaboration with Guoyang Luo to submit a clinical protocol entitled “Continuous monitoring of the placental oxygenation during pregnancy” to the Howard University IRB. The primary objective of the protocol is to study and evaluate the oxygenation level of the placenta in order to standardize and correlate the oxygenation data with placenta pathology and pregnancy outcomes. The protocol will recruit up to 1,000 pregnant women from underserved communities over the course of five years. Also, we are developing an analysis algorithm that evaluates the behavior of placental cells in consideration of various oxygen levels, using the dynamic full-field optical coherence tomography (DFFOCT) system in parallel with measurement of placental oxygenation using the NIRS device.
We are developing an algorithm designed to analyze changes in the dynamic activity (frequency and magnitude of cells) within a cell and calculate a mean frequency representing a weighted frequency. As a preliminary experiment, we evaluated the viability status of HeLa cells, an immortal human cell line widely used in cell research, from alive to dead. With the developed algorithm, the dynamic activity of cells was quantitatively and clearly distinguishable according to changes in their viability status [Reference 5]. From these results, we believe that DFFOCT can be used to analyze changes in cellular dynamic activity depending on the nutrient and oxygen saturation contained in placental cells. We hypothesize that there is a relationship between the dynamic activity of placental cells and potential neuro-developmental disorders. For the next study, we will analyze the dynamic activity of the placenta cells, taking into account various oxygen levels in the placenta. To this end, we plan to create a special sample chamber to control and maintain the oxygen concentration in the cells while conducting the experiment.
Additional Funding
- Bench to Bedside Award 345 (2016): “Mirror neuron network dysfunction as an early biomarker of neurodevelopment” (ongoing)
- Human Placenta Project—NICHD (2016, ongoing)
Publications
- Khaksari K, Smith EG, Miguel HO, Zeytinoglu S, Fox N, Gandjbakhche AH. An fNIRS study of brain lateralization during observation and execution of a fine motor task. Front Hum Neurosci 2022 15:798870.
- Dashtestani H, Miguel HO, Condy EE, Zeytinoglu S, Millerhagen JB, Debnath R, Smith E, Adali T, Fox NA, Gandjbakhche AH. Structured sparse multiset canonical correlation analysis of simultaneous fNIRS and EEG provides new insights into the human action-observation network. Sci Rep 2022 12:6878.
- Mah AJ, Nguyen T, Ghazi Zadeh L, Shadgan A, Khaksari K, Nourizadeh M, Zaidi A, Park S, Gandjbakhche AH, Shadgan B. Optical monitoring of breathing patterns and tissue oxygenation: a potential application in COVID-19 screening and monitoring. Sensors (Basel) 2022 22:7274.
- Nguyen T, Khaksari K, Khare SM, Park S, Anderson AA, Bieda J, Jung E, Hsu CD, Romero R, Gandjbakhche AH. Non-invasive transabdominal measurement of placental oxygenation: a step toward continuous monitoring. Biomed Opt Express 2021 12(7):4119–4130.
- Park S, Nguyen T, Benoit E, Sackett DL, Garmendia-Cedillos M, Pursley R, Boccara C, Gandjbakhche A. Quantitative evaluation of the dynamic activity of HeLa cells in different viability states using dynamic full-field optical coherence microscopy. Biomed Opt Express 2021 112(10):6431–6441.
Collaborators
- Franck Amyot, PhD, Center for Neuroscience and Regenerative Medicine, Uniformed Services University of the Health Sciences (USUHS), Bethesda, MD
- Mehran Armand, PhD, The Johns Hopkins University, Baltimore, MD
- Claude Boccara, PhD, École Supérieure de Physique et de Chimie Industrielles, Paris, France
- Nathan Fox, PhD, University of Maryland, College Park, MD
- Andrea Gropman, MD, Children's National Health System, Washington, DC
- Jay Knutson, PhD, Laboratory of Molecular Biophysics, NHLBI, Bethesda, MD
- Guoyan Luo, MD, PhD, Howard University Hospital, Washington, DC
- Tom Pohida, MS, Division of Computational Bioscience, CIT, NIH, Bethesda, MD
- Randall Pursley, MSc, Signal Processing and Instrumentation Section, CIT, NIH, Bethesda, MD
- Jessica C. Ramella-Roman, PhD, Florida International University, Miami, FL
- Roberto Romero-Galue, MD, DMedSci, Perinatology Research Branch, NICHD, Detroit, MI
- Dan Sackett, PhD, Division of Basic and Translational Biophysics, NICHD, Bethesda, MD
- Babak Shadgan, MD, MSc, PhD, University of British Columbia, Vancouver, Canada
- Audrey Thurm, PhD, Pediatrics & Developmental Neuropsychiatry Branch, NIMH, Bethesda, MD
- Bruce Tromberg, PhD, Section on Biomedical Optics, NICHD, Bethesda, MD
- Eric Wassermann, MD, Cognitive Neuroscience Section, NINDS, Bethesda, MD
Contact
For more information, email gandjbaa@mail.nih.gov or visit https://www.nichd.nih.gov/research/atNICHD/Investigators/gandjbakhche.