Molecular Genetics of Heritable Human Disorders
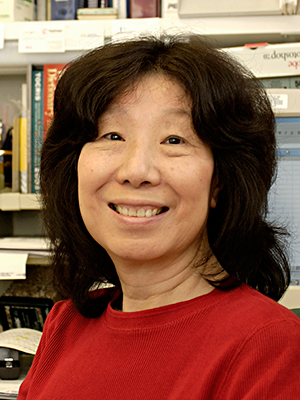
- Janice Y. Chou, PhD, Head, Section on Cellular Differentiation
- Irina Arnaoutova, PhD, Staff Scientist
- Lisa Zhang, PhD, Staff Scientist
- Sudeep Gautam, PhD, Visiting Fellow
- Javier Anduaga, BS, Technical Intramural Research Training Award Fellow
- Larissa Baeva, MS, Contract Technician
- Hung-Dar Chen, PhD, Contract Technician
- Cheol Lee, PhD, Contract Technician
We conduct research to delineate the pathophysiology and develop novel therapies for the three subtypes of type I glycogen storage disease (GSD-I), GSD-Ia, GSD-Ib, and GSD-Irs (GSD-I–related syndrome). GSD-Ia is caused by a deficiency in glucose-6-phosphatase-α (G6Pase-α or G6PC), GSD-Ib by a deficiency in the G6P transporter (G6PT or SLC37A4), and GSD-Irs, also known as severe congenital neutropenia syndrome type 4, by a deficiency in G6Pase-β. G6Pase-α and G6Pase-β are endoplasmic reticulum (ER)–bound G6P hydrolases, with active sites lying inside the lumen, which depend upon G6PT to translocate G6P from the cytoplasm into the ER lumen. The G6PT/G6Pase-α complex maintains interprandial glucose homeostasis, while the G6PT/G6Pase-β complex maintains energy homeostasis and functionality of neutrophils. GSD-Ia and GSD-Ib patients manifest a common metabolic phenotype of impaired glucose homeostasis not shared by GSD-Irs. GSD-Ib and GSD-Irs patients manifest a common myeloid phenotype of neutropenia and myeloid dysfunction not shared by GSD-Ia. Inactivation of G6PT or G6Pase-β in neutrophils leads to enhanced apoptosis, which underlies neutropenia in GSD-Ib and GSD-Irs. A deficiency in either G6PT or G6Pase-β in neutrophils prevents recycling of glucose from the ER to the cytoplasm, leading to the impaired energy homeostasis that underlies neutrophil dysfunction in GSD-Ib and GSD-Irs. There is no cure for GSD-Ia, GSD-Ib, or GSD-Irs. Animal models of the three disorders are available and are being exploited to both delineate the disease more precisely and develop new treatment approaches, including gene therapy. We generated efficacious G6Pase-α–expressing and G6PT–expressing recombinant adeno-associated virus (rAAV) vectors and provided a proof-of-principle gene therapy in murine GSD-Ia and GSD-Ib that is safe, efficacious, and appropriate for entering clinical trials. In 2018, a human G6Pase-α–expressing rAAV vector developed in this laboratory (US patent number: 9,644,216; European patent number: EP3074510) was selected for the phase I/II clinical trial for human GSD-Ia (ClinicalTrials.gov Identifier: NCT03517085).
Sirtuin signaling controls mitochondrial function in GSD-Ia.
Mitochondrial dysfunction has been implicated in GSD-Ia, but the underlying mechanism and its contribution to hepatocellular adenoma and carcinoma (HCA/HCC) development remain unclear. We showed that hepatic G6Pase-α deficiency leads to downregulation of SIRT1 signaling, which underlies defective hepatic autophagy in GSD-Ia. SIRT1 is an NAD–dependent deacetylase that can deacetylate and PGC-1α, a master regulator of mitochondrial integrity, biogenesis, and function. We hypothesized that downregulation of hepatic SIRT1 signaling in G6Pase-α–deficient livers impairs PGC-1α activity, leading to mitochondrial dysfunction. Using liver-specific G6pc-knockout (L-G6pc–/–) mice, we showed that the G6Pase–deficient livers display defective PGC-1α signaling, reduced numbers of functional mitochondria, and impaired oxidative phosphorylation. Overexpression of hepatic SIRT1 restores PGC-1α activity, normalizes the expression of electron transport chain components, and increases mitochondrial complex IV activity. We previously showed that restoration of hepatic G6Pase-α expression normalizes SIRT1 signaling. More recently, we showed that restoration of hepatic G6Pase-α expression also restores PGC-1α activity and mitochondrial function. We also demonstrated that HCA/HCC lesions found in G6Pase-α–deficient livers display marked mitochondrial and oxidative DNA damage. Taken together, our study shows that downregulation of hepatic SIRT1/PGC-1α signaling underlies mitochondrial dysfunction and that oxidative DNA damage incurred by damaged mitochondria may contribute to HCA/HCC development in GSD-Ia [Reference 1].
Hepatic G6Pase-α deficiency leads to metabolic reprogramming in GSD-Ia.
We had shown that hepatic G6Pase-α deficiency–mediated steatosis leads to the defective autophagy that is frequently associated with carcinogenesis. We showed that hepatic G6Pase-α deficiency also leads to enhancement of hepatic glycolysis and the hexose monophosphate shunt (HMS), which can contribute to hepatocarcinogenesis. The enhanced hepatic glycolysis is reflected in augmented lactate accumulation, increased expression of many glycolytic enzymes, and elevated expression of c-Myc, which stimulates glycolysis. The increased HMS is reflected in increased G6P dehydrogenase activity, elevated production of NADPH, and reduced glutathione. We showed that restoration of hepatic G6Pase-α expression normalizes both glycolysis and HMS in GSD-Ia. Moreover, the HCA/HCC lesions in L-G6pc–/– mice exhibit elevated levels of hexokinase 2 and the M2 isoform of pyruvate kinase, which play an important role in aerobic glycolysis and cancer cell proliferation. Taken together, hepatic G6Pase-α deficiency causes metabolic reprogramming, leading to enhanced glycolysis and elevated HMS, which, along with impaired autophagy and mitochondrial dysfunction, can contribute to HCA/HCC development in GSD-Ia [Reference 3].
Gene therapy prevents hepatic tumor development in murine GSD-Ia at the tumor-developing stage.
The hallmarks of GSD-Ia are impaired glucose homeostasis and long-term risk of HCA/HCC. Currently, there is no therapy to address HCA/HCC in GSD-Ia. We previously developed an rAAV vector–mediated gene therapy for GSD-Ia and showed that rAAV-G6PC–treated G6pc–/– mice expressing 3% or more of normal hepatic G6Pase-α activity maintain glucose homeostasis and do not develop HCA/HCC. However, it remains unclear whether G6PC gene transfer at the tumor-developing stage of GSD-Ia can prevent tumor initiation or abrogate the pre-existing tumors. Using L-G6pc–/– mice that develop HCA/HCC, we showed that treating the mice at the tumor-developing stage with rAAV-G6PC restores hepatic G6Pase-α expression, normalizes glucose homeostasis, and prevents de novo HCA/HCC development. The rAAV-G6PC treatment also normalizes defective hepatic autophagy and corrects metabolic abnormalities. However, gene therapy cannot restore G6Pase-α expression in the HCA/HCC lesions and fails to abrogate any pre-existing tumors.
The major regulators of hepatic glucose metabolism are the glucocorticoids that promote gluconeogenesis. We examined the expression of 11 β-hydroxysteroid dehydrogenase type-1 (11βHSD1), which mediates local glucocorticoid activation by converting inert cortisone (in human) and dehydrocorticosterone (in rodents) into active cortisol and corticosterone, respectively. We showed that the expression of 11βHSD1 is downregulated in HCA/HCC lesions, leading to impairment in glucocorticoid signaling, which is critical for gluconeogenesis activation. This suggests that downregulation of local glucocorticoid action in the HCA/HCC lesions may suppress gene therapy–mediated G6Pase-α restoration. Collectively, our data show that rAAV–mediated gene therapy can prevent de novo HCA/HCC development in L-G6pc–/– mice at the tumor developing stage, but that it cannot reduce any pre-existing tumor burden [Reference 4].
An evolutionary approach to optimizing G6Pase-α activity for gene therapy of GSD-Ia
GSD-Ia is characterized by impaired glucose homeostasis with the hallmark of hypoglycemia following a short fast. Previously, we developed rAAV vectors expressing either the wild-type (WT) (rAAV-hG6PC-WT) or codon-optimized (co) (rAAV-co-hG6PC) human (h) G6Pase-α. We showed that G6pc–/– mice treated with either rAAV-hG6PC-WT or rAAV-co-hG6PC maintain glucose homeostasis and do not develop HCA/HCC if they restore 3% or more of normal hepatic G6Pase-α activity. The codon-optimized vector, which has a higher potency, is currently being used in a phase I/II clinical trial for human GSD-Ia (NCT 03517085). While routinely used in clinical therapies, codon-optimized vectors may not always be optimal. Codon-optimization can impact RNA secondary structure, change RNA/DNA protein binding sites, affect protein conformation and function, and alter posttranscriptional modifications that may reduce potency or efficacy. We therefore sought to develop alternative approaches that could improve the expression yet minimize the impact of sequence changes resulting from broad codon optimization. The human, dog, mouse, and rat G6Pase-α share 87–91% sequence identity. Intriguingly, in vitro expression assays have routinely shown that the canine G6Pase-α isozyme is significantly more active than hG6Pase-α. We therefore expanded our analysis to compare G6PC genes across the evolutionary tree, seeking potential codon changes that could enhance enzymatic activity of hG6Pase-α. We identified a Ser-298 to Cys-298 substitution naturally found in canine, mouse, rat, and several primate G6Pase-α isozymes, which, when incorporated hG6Pase-α sequence, markedly enhanced enzymatic activity. Using G6pc–/– mice, we showed that the efficacy of the rAAV-hG6PC-S298C vector was threefold higher than that of the rAAV-hG6PC-WT vector. The rAAV-hG6PC-S298C vector with increased efficacy, that minimizes the potential problems associated with codon-optimization, offers a valuable vector for clinical translation in human GSD-Ia [Reference 5].
Additional Funding
- The Children's Fund for Glycogen Storage Disease Research, 2017
- CRISPR Therapeutics, (Cambridge, MA) under a cooperative research and development agreement (CRADA)
Publications
- Cho JH, Kim GY, Mansfield BC, Chou JY. Sirtuin signaling controls mitochondrial function in glycogen storage disease type Ia. J Inherit Metab Dis 2018;41:997-1006.
- Chou JY, Cho JH, Kim GY, Mansfield BC. Molecular biology and gene therapy for glycogen storage disease type Ib. J Inherit Metab Dis 2018;41:1007-1014.
- Cho JH, Kim GY, Mansfield BC, Chou JY. Hepatic glucose-6-phosphatase-a deficiency leads to metabolic reprogramming in glycogen storage disease type Ia. Biochem Biophys Res Commun 2018;498:925-931.
- Cho JH, Lee YM, Starost, MF, Mansfield BC, Chou JY. Gene therapy prevents hepatic tumor development in glycogen storage disease type Ia mice at the tumor-developing stage. J Inherit Metab Dis 2019;42:459-469.
- Zhang L, Cho JH, Arnaoutova I, Mansfield BC, Chou JY. An evolutionary approach to optimizing glucose-6-phosphatase-alpha enzymatic activity for gene therapy of glycogen storage disease type Ia. J Inherit Metab Dis 2019;42:470-479.
Collaborators
- Alessandra Eva, PhD, Istituto Giannina Gaslini, Genova, Italy
- Hyun Sik Jun, PhD, Korea University, Seoul, South Korea
- Youngmok Lee, PhD, University of Connecticut School of Medicine, Farmington, CT
- Matthew F. Starost, PhD, Diagnostic & Research Services Branch, Division of Veterinary Resources, NIH, Bethesda, MD
- David A. Weinstein, MD MSc, University of Connecticut School of Medicine, Farmington, CT
Contact
For more information, email chou@helix.nih.gov or visit https://irp.nih.gov/pi/janice-chou.