Neuronal Circuits Controlling Behavior: Genetic Analysis in Zebrafish

- Harold Burgess, PhD, Head, Section on Behavioral Neurogenetics
- Ashwin A. Bhandiwad, PhD, Postdoctoral Fellow
- Eric J. Horstick, PhD, Postdoctoral Fellow
- Abhignya Subedi, PhD, Postdoctoral Fellow
- Kathryn M. Tabor, PhD, Postdoctoral Fellow
- Gregory Marquart, BSc, Graduate Student
- Yared Bayleyen, BSc, Postbaccalaureate Fellow
- Trevor S. Smith, BSc, Postbaccalaureate Fellow
- Jennifer L. Strykowski, MSc, Zebrafish Technician
Our goal is to understand how, under diverse environmental contexts, the nervous system selects appropriate behavioral actions in a way that best satisfies internal motivational objectives. We use the zebrafish as a model because, in the larval stage, its brain exhibits the basic architecture of the vertebrate brain but is much less complex than the mammalian brain. Despite the relative simplicity of the nervous system, larvae have a sophisticated repertoire of sensory-guided and internally driven behaviors. Furthermore, the optical clarity of the embryo facilitates visualization of individual neurons and their manipulation with genetic techniques. Behavior in larvae is innate and therefore exhibits minimal variability between fish. Subtle alterations in behavior can therefore be robustly scored, making it possible to quickly assess the contribution of identified neurons to a variety of motor behaviors.
We focus on two aspects of behavioral regulation: the mechanisms by which sensory context regulates behavioral decisions (Reference 1) and pathways that sustain changes in behavioral state (Reference 2). In addition, we are developing a suite of genetic tools and behavioral assays to probe the nexus between neuronal function and behavior at single-cell resolution (References 3–5). The neuronal connections that allow the brain to integrate sensory and internal-state information are established through genetic interactions during development. We aim to identify genes and neurons that are required for the functional development of such connections. In vertebrates, neuronal circuits situated in the brainstem form the core of the locomotor control network and are responsible for balance, posture, motor control, and arousal. Accordingly, many neurological disorders stem from abnormal formation or function of brainstem circuits. Insights into the function of brainstem circuits in health and disease have come from genetic manipulation of neurons in zebrafish larvae in combination with computational analysis of larval behavior.
Molecular identification of neurons that mediate prepulse inhibition
Startle responses are rapid reflexes that are triggered by sudden sensory stimuli; they help animals to defend against and escape from potentially threatening stimuli. In both fish and mammals, startle responses are initiated by giant reticulo-spinal neurons in the medulla, which receive short-latency sensory input from diverse sensory modalities. Although highly stereotyped, startle responses are nevertheless modulated by sensory context and behavioral state and are therefore an excellent system in which to understand how such information is integrated with behavioral selection.
In mammals, including humans, the startle response to a strong auditory stimulus can be inhibited by pre-exposure to a weak acoustic 'prepulse.' This form of startle modulation, termed prepulse inhibition, is diminished in several neurological conditions, including schizophrenia. The precise pattern of neuronal connections that enable prepulse inhibition is not known, yet understanding the mechanism of prepulse inhibition would permit rational investigation into the mechanism by which schizophrenia risk factors influence neuronal circuitry. Vibrational stimuli trigger rapid-escape swims in zebrafish, which are mediated by giant reticulo-spinal neurons, in a manner similar to the central neurons controlling startle responses in mammals. Escape swims are suppressed by pre-exposure to a prepulse, allowing us to apply the powerful suite of genetic tools available in zebrafish to identify neurons that mediate prepulse inhibition.
To identify a transgenic zebrafish line that genetically labels neurons required for prepulse inhibition, we conducted a circuit-breaking screen. In this screen, we first generated a library of neuron-specific Gal4–enhancer trap lines marking distinct populations of neurons in the brain; we then ablated the neurons in each enhancer trap line before testing for prepulse inhibition. We identified a transgenic line, y252, that labeled a discrete population of neurons in the hindbrain whose ablation or opto-genetic inhibition led to dysregulation of prepulse inhibition. We discovered that the neurons genetically labeled in y252 are specified by the gene Gsx1, a transcription factor previously implicated in differentiation of both excitatory and inhibitory interneurons in the spinal cord. Gsx1 was expressed in the mouse brainstem in the proliferative zone, which gives rise to regions previously implicated in prepulse inhibition. We obtained Gsx1 knockout mice and performed behavioral testing. Knockout mice showed normal startle sensitivity but a strong reduction in prepulse inhibition. The results thus show that Gsx1 expression defines neurons that are required for prepulse inhibition across vertebrate species. Given that prepulse inhibition is abnormal in neuropsychiatric disorders with developmental origins, including schizophrenia and autism, our work will help identify and probe fundamental defects in circuitry that is abnormal in these conditions (Reference 1).
A major outstanding question remains the specific identity of the Gsx1 neurons responsible for prepulse inhibition, given that these neurons are located in several regions of the brain. To tackle this question, we developed new methods for monitoring and manipulating subgroups of Gsx1 neurons. First, we use volumetric calcium imaging to simultaneously visualize the activity in thousands of Gsx1 neurons during a prepulse inhibition paradigm. At the same time, we record tail movement responses to startle stimuli, with and without prepulse exposure. This is enabling us to locate specific Gsx1 neurons whose activity correlates strongly with behavioral prepulse inhibition. To demonstrate that such neurons are causally related to prepulse inhibition, we established an intersectional genetic method to ablate subgroups of Gsx1 neurons, by developing a library of Cre lines (Figure 1) and a UAS vector that requires both Gal4 and Cre expression in the same cell for reporter expression (UAS:KillSwitch).
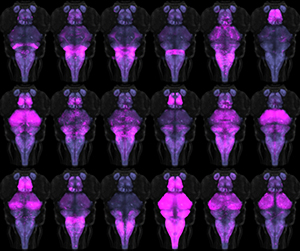
Click image to enlarge.
Figure 1. Cre transgenic lines for intersectional genetic targeting
Montage of 18 transgenic and enhancer trap lines that express Cre in discrete subsets of neurons. A UAS:Switch transgenic line ensures that only neurons expressing both Gal4 and Cre are labeled by a reporter gene.
Pharmacological experiments in mammals suggest that prepulse inhibition is likely to be mediated by several mechanisms with overlapping temporal profiles. Our genetic experiments support this idea. Inactivation of Gsx1 neurons in zebrafish leads only to a deficit in prepulse inhibition at inter-stimulus intervals between prepulse and startle stimulus of greater than 100 ms, indicating that a separate group of neurons instantiate prepulse inhibition at short inter-stimulus intervals. We therefore established a new circuit-breaking screen to locate these neurons.
Tools for analyzing neuronal circuits that control behavior
We previously described a new method for restricting transgene expression to the nervous system, by incorporating a neuronal-restrictive silencing element. Using this method, we constructed a library of 240 Gal4 enhancer-trap transgenic fish with unique and restricted patterns of neuronal expression. By performing high-resolution brain imaging on more than 300 larvae—including 80 of these lines and 29 other transgenic lines—and applying software developed for human brain imaging, we aligned the brain scans to a common reference, so that the expression in any of these lines can be compared at cellular-level resolution (Figure 2). This database, with associated Brain Browser software, formed the basis for a new atlas of the larval brain, a powerful resource for neurobiological studies (Reference 3).

Click image to enlarge.
Figure 2. A transgene expression atlas for zebrafish
We imaged more than 100 transgenic lines at high resolution and computationally registered images to a common reference brain, so that expression patterns could be overlaid. New Brain Browser software allows transgenic lines that label selected brain regions to be quickly identified and permits prediction of transgenic lines that co-express in the same cell populations. Users browse the atlas by simultaneously viewing horizontal (a), transverse (b) and sagittal (c) views of brain sections or projections (a–b), which display expression data for co-registered transgenic lines. In addition, a 3D projection view helps reveal the spatial relationships of brain regions and expression patterns (d).
A unique feature of brain imaging in zebrafish is the possibility of visualizing the overall architecture of the brain, while simultaneously recording the position and morphology of constituent labeled neurons. Thus, a particular challenge for deformable brain registration in larval zebrafish is to achieve highly precise global registration, without severely distorting the shape of individual cells. In the first release of our Brain Browser, we used an elastic brain registration algorithm that co-aligned brains with a precision of about 1 cell diameter but also produced salient abnormalities in cell morphology in several brain regions. More recently, we calibrated parameters for the symmetric diffeomorphic normalization algorithm in ANTs (Advanced Normalization Tools) and found that it was possible to achieve similar, or better precision, without sacrificing cell morphology (Figure 3). In fact, multi-channel registration with ANTs allowed us to integrate our database of transgene expression patterns with an independent zebrafish brain atlas, Z-Brain, that contains additional gene expression patterns and neuroanatomical annotations (Reference 4).

Click image to enlarge.
Figure 3. Improved brain registration for larval zebrafish
We compared several algorithms for deformable image registration to optimize brain alignment in larval zebrafish. Our previous studies employed elastic registration using a b-spline–based transformation, which resulted in distortion of cell morphology in several brain regions, such as the caudal hypothalamus (a), and imprecise registration in other parts of the brain, including the tectal neuropil (b). In contrast, symmetrical diffeomorphic transformation using ANTs better preserved cell morphology (c) and increased the precision of registration in areas such as the tectal neuropil (d).
The more than 100 lines included in the Brain Browser database together provide a way to transgenically target at least 70% of neurons in the larval brain. Most of these lines express Gal4 allowing activation of reporters downstream of a UAS (upstream activating sequence) element. We therefore also worked to improve existing UAS reporters for ablating and activating neurons. Specifically, we developed new reagents and protocols that allow for highly efficient neuronal ablation or for sensitizing neurons to excitatory input in the context of their normal neuronal functions (Reference 5). Together, these genetic tools allow us to target highly specific neurons within the larval brain for visualizing activity or altering function, helping us and others decode their role within neuronal circuits.
Additional Funding
- US Israel Binational Fund 2013433 (2014): Ongoing, with Yoav Gothilf, Tel Aviv University
Publications
- Tabor K, Bergeron SA, Horstick EJ, Jordan DC, Aho V, Porkka-Heiskanen T, Haspel G, Burgess HA. Direct activation of the Mauthner cell by electric field pulses drives ultrarapid escape responses. J Neurophysiol 2014; 112:834-844.
- Bergeron SA, Carrier N, Li GH, Ahn S, Burgess HA. Gsx1 expression defines neurons required for prepulse inhibition. Mol Psychiatry 2015; 20:974-985.
- Marquart GD, Tabor KM, Brown M, Strykowski JL, Varshney GK, LaFave MC, Mueller T, Burgess SM, Higashijima I, Burgess HA. A 3D searchable database of transgenic zebrafish Gal4 and Cre lines for functional neuroanatomy studies. Front Neural Circuits 2015; 9:78.
- Horstick EJ, Jordan DC, Bergeron SA, Tabor KM, Serpe M, Feldman B, Burgess HA. Increased functional protein expression using nucleotide sequence features enriched in highly expressed genes in zebrafish. Nucleic Acids Res 2015; 43:e48.
- Horstick EJ, Tabor KM, Jordan DC, Burgess HA. Genetic silencing and selective activation using nitroreductase and TTX insensitive channels. Methods Mol Biol: Zebrafish 2015; in press.
Collaborators
- Kevin Briggman, PhD, Circuit Dynamics and Connectivity Unit, NINDS, Bethesda, MD
- Benjamin Feldman, PhD, Zebrafish Core Facility, NICHD, Bethesda, MD
- Yoav Gothilf, PhD, Tel-Aviv University, Tel-Aviv, Israel
- Shin-ichi Higashijima, PhD, National Institutes of Natural Sciences, Aichi, Japan
- Michael J. Iadarola, PhD, Warren Grant Magnuson Clinical Center, NIH, Bethesda, MD
- Thomas Mueller, PhD, Kansas State University, Manhattan, KS
- Ralph Nelson, PhD, Basic Neurosciences Program, NINDS, Rockville, MD
- Forbes Porter, MD, PhD, Program in Developmental Endocrinology and Genetics, NICHD, Bethesda, MD
- Mihaela Serpe, PhD, Program in Cellular Regulation and Metabolism, NICHD, Bethesda, MD
Contact
For more information, email haroldburgess@mail.nih.gov or visit http://ubn.nichd.nih.gov.