Point of Care and Wearable Biophotonics for Characterizing Tissue Composition and Metabolism
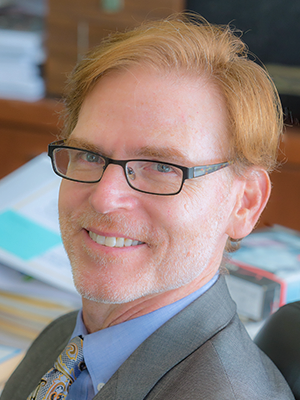
- Bruce Tromberg, PhD, Head, Section on Biomedical Optics, Director of the National Institute of Biomedical Imaging and Bioengineering
- Timothy Quang, PhD, Staff Scientist
- Brian Hill, MS, Biomedical Engineer
- Yun He, PhD, Postdoctoral Fellow
- Sully Chen, BS, Postbaccalaureate Fellow
- Amir Sadikov, BS, Postbaccalaureate Fellow
- Kathryn Jaroszynski, Student Fellow
By advancing models, methods, and devices that utilize the interaction of light with biological tissue, we strive to develop non-invasive techniques that can help guide therapy and aid in clinical decision making. The techniques are used to perform real-time quantitative measurements of clinically relevant information, including tissue blood flow, oxygen extraction, and body/tissue composition. Our research seeks to move such technologies from ‘bench to bedside,’ where they can be applied to clinical problems, including vascular and metabolic disease.
Non-invasive optical imaging technology for characterization of tissue hemodynamics and composition
Aberrations in tissue hemodynamics (i.e., blood flow, oxygenation, and oxygen metabolism) and tissue composition are observed in a wide variety of diseases, including cancer, cardiovascular disease, diabetes, and neurodegenerative disorders. In all such conditions, techniques that can characterize tissue hemodynamics and composition can improve strategies for early diagnosis, screening, and treatment-response monitoring.
There are several clinically accepted ways to assess cardiovascular function, specifically pertaining to tissue metabolism and composition. Techniques such as functional magnetic resonance imaging (fMRI) and positron emission tomography (PET) have been used for measuring blood flow through tissue. Additionally, MRI and dual X-ray absorptiometry (DXA) can be used to determine body composition and distinguish between lean and soft tissue. While the techniques can provide comprehensive cardiovascular assessments, they are also time- and resource-intensive and can only be accessed in a hospital setting. As a result, patients typically undergo such assessments only after the onset of severe symptoms. At-home technologies, while more accessible to the general public, are limited to relatively crude cardiovascular assessments such as heart rate, oxygen saturation, and blood pressure. As alterations in vascular health occur gradually over time, there is a need for technologies that can comprehensively assess tissue hemodynamics and composition at the point of care.
To characterize tissue hemodynamics or composition in portable form factors, near-infrared spectroscopy (NIRS) techniques have emerged as lower-cost and non-invasive alternatives. Techniques such as diffuse optical spectroscopic imaging (DOSI) or spatial frequency domain imaging (SFDI) can quantify the concentration of hemoglobin, water, and bulk lipids. In addition to the compositional information that can be obtained, continuous measurements with such techniques can also assess tissue hemodynamics in terms of the delivery and consumption of oxygen by observing changes in oxy- and deoxy-hemoglobin concentration. While the techniques employ similar principles to measure similar information, they differ in terms of the field of view and the depth of tissue interrogated. Another subset of NIRS techniques can quantify blood flow, which is necessary to more accurately characterize tissue metabolic activity. Technologies such as laser speckle imaging (LSI), laser Doppler flowmetry (LDF), and diffuse correlation spectroscopy (DCS) all measure fluctuations in intensity caused by light-scattering events from moving particles such as red blood cells, in order to provide quantitative measure of blood flow.
These diverse optical technologies are promising candidates to provide more comprehensive assessments of tissue metabolism and composition in a range of different measurement configurations and enable our group to select optical modalities that best suit a given clinical context. We are currently working on translating the biophotonics technologies into a clinical setting and characterizing the tissue composition and metabolism in several patient cohorts with various disease states.
We are performing the current studies in collaboration with various groups in the NIH Clinical Center to evaluate changes in tissue metabolism and composition in response to different therapeutic interventions:
NCT04595773 – COVID-19 – Chronic Adaptation and Response to Exercise (COVID-CARE): a randomized controlled trial
The study, led by Leighton Chan, will evaluate whether a rehabilitation exercise program can help people recovering from COVID-19. Standard clinical cardiovascular-functions tests to evaluate recovery will be compared with optically obtained biomarkers.
NCT03538639 – Vascular Disease Discovery Protocol
Led by Manfred Boehm, the study follows individuals with diseases of the heart and/or blood vessels. Optical signals will be characterized in vascular disease and compared with standard clinical health tests.
Optical characterization of vascular health in sickle cell disease
Given the impairments to microvascular flow and endothelial dysfunction associated with sickle cell disease (SCD), advanced quantitative NIRS is an attractive candidate to provide comprehensive hemodynamic evaluations in point-of-care settings. There are limited studies evaluating NIRS devices in SCD applications. We are optically characterizing tissue composition, metabolism, and perfusion in a cohort of SCD patients being treated with Mitapivat, a pyruvate kinase activator. While on Mitapivat, we expect improvement in the patients’ red blood cell metabolism, which reduces the occurrence of hemoglobin (Hb) sickling and also improves their patients' survivability. This can be observed in blood chemistry as an increase in blood hemoglobin levels in addition to a reduction in hemolytic markers such as lactate dehydrogenase (LDH) and reticulocyte count.
Our group is assessing the sensitivity of various optical devices to hemodynamic changes induced by these treatments and evaluating whether the changes correlate with what is observed from blood chemistry. The study is being performed in collaboration with the NHLBI sickle cell branch led by Swee Lay Thein. Following an initial baseline visit, patients are started on the study drug and return for regular, follow-up visits according to a pre-set schedule. NIRS measurements are acquired at each of these visits, including at the baseline visit. For the NIRS component of the study, hemodynamics from the skin, fingertip, and skeletal muscle tissue compartments are non-invasively acquired by multiple NIRS modalities during a brachial cuff occlusion measurement (Figure 1). In this observational, proof-of-concept study, we showed that it is feasible to perform all the different measurements within an hour, with minimal discomfort to the subjects.
Figure 1. Sickle cell disease (SCD) measurement setup
Optical imaging setup for the SCD study. Several NIRS modalities are deployed for assessing hemodynamic changes during the course of treatment. The bounding box (right) features a closer view of the optical probes that capture hemodynamics in the fingertip (A), skin (B), and skeletal muscle (C).
Patients enrolled in the study undergo a brachial cuff occlusion at each study visit. Prior literature cited the reactive hyperemic response following the end of a brachial cuff occlusion as an index of endothelial function, using modalities such as peripheral arterial tonometry. A larger hyperemic response is indicative of better endothelial function. Figure 2 summarizes some of the optical data during an occlusion from a SCD patient who exhibited an expected response to the Mitapivat treatment. Figure 2a compares the skeletal muscle oxyhemoglobin (O2Hb) response at the baseline visit and the first study visit in which the patient had a more than 1 g/dL increase in blood Hb level. Both responses were normalized by their respective mean O2Hb concentration during the three-minute baseline time for ease of comparison. During the response visit, we can observe a larger hyperemic response in the O2Hb trace, which can be quantified as the magnitude of the difference between the O2Hb levels at the end of the occlusion and at the peak of the hyperemic response (ΔO2Hb). Figure 2b compares the blood perfusion data acquired from diffuse reflectance spectroscopy (DRS) in the fingertip during the baseline and response visits. As was observed in the skeletal muscle data, we can observe a greater hyperemic response during the response visit, which we can quantify in terms of the area under the curve (AUC) of the respective curves. The data suggest that there is an observable change in the vascular reactivity as a result of the Mitapivat treatment.
Figure 2. Occlusion response at baseline and during therapy
a. Comparison of skeletal muscle O2Hb response at the baseline visit and the first study visit in which the patient had a greater than 1 g/dL increase in blood Hb level. Both responses have been normalized by their respective mean O2Hb concentration during the three-minute baseline time for ease of comparison. During the response visit, we can observe a larger hyperemic response in the O2Hb trace, which can be quantified as the magnitude of the difference between the O2Hb levels at the end of the occlusion and at the peak of the hyperemic response (ΔO2Hb).
b. Comparison of the blood perfusion data acquired from diffuse reflectance spectroscopy (DRS) in the fingertip during the baseline and response visits. As was observed in the skeletal muscle data, we can observe a greater hyperemic response during the response visit. The data begin to suggest that there is an observable change in the vascular reactivity as a result of the Mitapivat treatment.
Figure 3 compares the FD-NIRS–derived optical hyperemic response to the LDH levels taken from blood draws at each available study visit (n = 14 visits). LDH has also been reported as a potential marker of nitric oxide (NO) bioavailability, which is a key component of endothelial function. In this initial dataset, there is a moderate, negative correlation (R2 = 0.61) between the ΔO2Hb and LDH levels, which would be expected, given that the lower LDH levels would signify greater bioavailability of NO and therefore improved endothelial function. As the study continues, we will incorporate additional data to further verify our findings.
Figure 3. Lactate dehydrogenase vs. delta-oxyhemoglobin
LDH from blood draws is compared to the FD-NIRS–derived hyperemic response for n=14 study visits. LDH is a potential marker of nitric oxide (NO) bioavailability, which is a key component of endothelial function. In this initial dataset, there is a moderate, negative correlation between the ΔO2Hb and LDH levels, which would be expected as lower LDH levels would signify greater bioavailability of NO and therefore improved endothelial function.
Development of a wearable point-of-care monitoring device for pediatric obstructive sleep apnea
Obstructive sleep apnea (OSA) is the most common type of sleep apnea, in which the blockage of the airway causes breathing to stop involuntarily for ten seconds or more throughout the night during sleep. When breathing stops, the oxygen level in the blood can drop to harmfully low levels. Pediatric obstructive sleep apnea (POSA) can be especially concerning, with several associated morbidities that can have long-term effects extending into adulthood, including adverse changes in cardiovascular, metabolic, and developmental health. Unfortunately, POSA remains largely under-diagnosed owing to a lack of education about symptoms and limited availability of sleep-medicine physicians. Early diagnosis and treatment are imperative to prevent many of the morbidities. Polysomnography (PSG), the current standard of care, is expensive, cumbersome, and resource-intensive. At-home sleep apnea tests (HSAT) are less expensive but are equally uncomfortable, prone to user error, and are less effective in children. There is a pressing need for an accurate, robust, unobtrusive alternative for monitoring POSA in the home.
NIRS is a non-invasive optical technique that can provide direct examination of tissue hemodynamics using near-infrared light in the range of 700 to 1000 nm. Compared with other well established brain imaging modalities, such as fMRI and PET, the technique has a higher temporal resolution (in order of milliseconds), and provides additional physiological information, including heart rate variability and respiration rate. Also, NIRS devices are smaller and can be built into a compact, inexpensive form factor, which is advantageous for ease-of-use and accessibility. NIRS instruments can tolerate subject motion to a greater extent than can fMRI. Such features make the technique ideally suited for studying children, particularly those with problems such as attention-deficit hyperactivity disorder (ADHD), which can make keeping still for long periods of time highly challenging. The relationships between NIRS parameters and traditional measurements taken during a sleep study are still largely unknown. NIRS could provide better prediction of health outcomes and a greater understanding of brain hypoxia during OSA events.
Figure 4. Cerebral hemodynamics during apnea
Cerebral oxyhemoglobin (O2Hb), deoxyhemoglobin (HHb), and nasal pressure during an obstructive apnea event. During apnea, cerebral HHb increases, reaching its maximum at the end of the event. As breathing resumes, cerebral HHb falls and O2Hb increases, signifying recovery from the hypoxia.
Initial measurements using the platform were obtained on adults recruited at the UC Irvine sleep center by Ruth Benca and Rami Khayat. Figure 4 shows NIRS signals for oxy- (O2Hb) and deoxy-hemoglobin (HHb) during a sample apnea event. The most pronounced feature is the rise in HHb during the interruption in respiration (nasal air flow obtained by PSG is shown in green). Based on this feature, we created an automated optical index by counting the number of HHb peaks occurring per hour. Sleep sessions were separated into 30 minute increments over which our optical index and the number of apnea and hypopnea events per hour (AHI) were determined. Apnea and hypopnea events are manually scored by a sleep technician following the sleep study. The Pearson’s correlation coefficient, a measure of the strength of linear association, was 0.88, indicating a strong correlation between our NIRS–based parameter and the traditional sleep metric (Figure 5).
Figure 5. AHI–DPI correlation
Scatterplot of DPI (deoxyhemoglobin peak index) vs. AHI (apnea hypopnea index) for contiguous thirty-minute intervals for each patient (separated by color) with the line of best fit (black).
The IRB protocol (NCT05052216) “Development of a Wearable Point of Care Monitoring Device for Pediatric Obstructive Sleep Apnea” was recently approved and will begin enrolling subjects at the NIH Clinical Center early next year in collaboration with Amir Gandjbakhche and Ashura Buckley. We will continue to investigate how optical signals change throughout sleep by comparing physiological signals derived from NIRS with data from PSG and how to develop future optical technologies to provide better patient care that can be used in a home setting. We will test the potential of NIRS technology to diagnose POSA and examine the response of the brain and tissue during apnea/hypopnea events.
Wearable laser-speckle imaging techniques
We are currently working to advance a modified form of laser-speckle imaging (LSI) known as affixed transmission speckle analysis (ATSA). The wearable device uses a single coherent light source and a camera sensor to capture two types of pulsatile waveform data, a waveform that is known as the photoplethysmographic (PPG) waveform, which is related to changes in blood volume, and the speckle plethysmographic (SPG) waveform, which is related to changes in blood flow. These related waveforms contain important information regarding cardiovascular health, with PPG commonly used in pulse oximetry as well as heart rate and heart-rate variation measurements. More advanced analyses of these signals can potentially aid in evaluating cardiovascular disease and produce markers of vascular stiffness. Additionally, the signals have the potential to act as a surrogate for continuous blood-pressure monitoring. Our lab is working to improve the ATSA technology by improving the accuracy and stability of the device over a larger range of flow speeds.
Blood pressure (BP) is one of the key markers of cardiovascular health, signaling the proper flow of blood from the heart to the body. While cuff-based methods for determining BP are reliable for standard clinical settings, they can be time-limited, allowing only intermittent measurements that are not easily integrated into everyday life. A continuous method of assessing BP would be highly beneficial for monitoring patients after any kind of medical intervention and could serve as a valuable predictor of future cardiovascular events. Recently, several approaches have evaluated pulse transit time (PTT) measurements as a means of estimating BP continuously. The techniques commonly employ ECG coupled with PPG waveforms and measure the time taken for an arterial pulse pressure wave to travel from the aortic valve to a peripheral site. SPG has been demonstrated to have better signal quality than PPG, which makes it an attractive candidate to evaluate in this context. Figure 6 shows preliminary data comparing PTT measurements based on PPG and SPG during a cold pressor test. The cold pressor test induces vasoconstriction and an increase in blood pressure by lowering the ambient temperature around a peripheral limb. While both methods display an inverse relationship with blood pressure, PPG PTT (Figure 6 left, R = -0.689) displays a more moderate correlation than does SPG PTT (Figure 6 right, R = -0.938). A study to further investigate SPG PTT as a marker for cuff-free continuous monitoring of BP is currently being developed.
Figure 6. Pulse transit time vs. blood pressure
Comparison of PTT (pulse transit time) using conventional PPG (photoplethysmography) (left) and SPG (speckle plethysmography) (right) during a cold pressor test. PTT based on SPG displays a higher correlation with measured blood pressure.
Development of a multimodal biosensor for screening and monitoring infectious diseases
The ongoing COVID-19 pandemic has been a taxing challenge to healthcare systems worldwide. A surge in cases can threaten to overwhelm hospitals' personnel and resources. The wide range and varying severities of COVID-19 symptoms further add to the challenge of managing the disease. While some cases of COVID-19 are mild and require no further intervention, more severe cases can involve major cardiorespiratory symptoms that could require a ventilator. Rapid, point-of-care tests are valuable for identifying patients who are infected with the virus; however, they do not serve as indicators for patient outcomes. A compact method to monitor patient health status and predict patient outcomes could improve our understanding of physiological impact of COVID-19 and alleviate potential strain on hospital resources.
The current standard of care to monitor patient health status revolves around vital-sign parameters such as heart rate, arterial oxygen saturation, respiration rate, and temperature. Some reports have examined personal health devices such as the Apple Watch, Oura Ring, and WHOOP, and the continuous health data they all collect, in order to develop models to identify symptomatic patients infected with COVID-19. However, the work still relies on the basic vital-sign parameters mentioned above, which only represent a small subset of information on how the body is functioning. Given the growing evidence of the negative impact of COVID-19 on the cardiovascular system, especially the microvasculature, characterization of microvascular health could further improve these predictive models.
NIRS is uniquely positioned to provide distinct information about the delivery and consumption of oxygen in compact form factors. Tissue oxygen saturation, which describes the oxygenation of blood at the tissue and capillary level, is one such metric that can offer valuable insight into microvascular function. In contrast, arterial oxygen saturation, which is normally reported with pulse oximetry, describes the oxygenation of blood coming from the arteries and is therefore an indicator only of oxygen supply. With a sufficiently high sampling rate, the PPG waveform can also be acquired, which enables NIRS to collect the same vital sign parameters as those collected by commercially available wearables.
We developed a multi-modal optical biosensor capable of real-time, continuous monitoring of tissue oxygenation in addition to vital-sign parameters such as heart rate, respiration rate, and temperature. Figure 7 demonstrates the performance of the biosensor in measuring deoxyhemoglobin changes during respiratory challenges compared with a commercial NIRS device. A pilot study to characterize performance of the biosensor in healthy adults has been approved (NCT05035420) and will soon start to enroll subjects. We believe that the device has the potential to provide continuous assessment of microvascular health, which can supplement current patient-monitoring tools and aid in the prediction of patient outcomes.
Figure 7. Validation of biosensor with a commercial NIRS device
Comparison of deoxyhemoglobin changes during breath hold and hyperventilation challenges as measured by the biosensor and a commercial NIRS system.
Additional Funding
- ”Development of a Wearable Point of Care Monitoring Device for Pediatric Obstructive Sleep Apnea,” NICHD Director’s Award, NIH IRP
Publications
- Lam J, Hill B, Quang T, Amelard R, Kim S, Yazdi H, Warren R, Cutler K, Tromberg B. Multi-modal diffuse optical spectroscopy for high-speed monitoring and wide-area mapping of tissue optical properties and hemodynamics. J Biomed Opt 2021;26:085002.
- Warren R, Bar-Yoseph R, Hill B, Reilly D, Chiu A, Radom-Aizik S, Cooper D, Tromberg B. Diffuse optical spectroscopic method for tissue and body composition assessment. J Biomed Opt 2021;submitted.
Collaborators
- Ruth Benca, MD, PhD, University of California, Irvine, CA
- Manfred Boehm, MD, Laboratory of Cardiovascular Regenerative Medicine, NHLBI, Bethesda, MD
- Ashura Buckley, MD, Office of the Clinical Director, NIMH, Bethesda, MD
- Leighton Chan, MD, MPH, Rehabilitation Medicine, Clinical Center, NIH, Bethesda, MD
- Ingemar Frederiksson, PhD, Perimed Instruments, Järfälla-Stockholm, Sweden
- Amir Gandjbakhche, PhD, Section on Analytical and Functional Biophotonics, NICHD, Bethesda, MD
- Ahmed Gharib, MD, Biomedical and Metabolic Imaging Branch, NIDDK, Bethesda, MD
- Ramy Khayat, MD, School of Medicine, University of California, Irvine, CA
- Thomas J. Pohida, MS, Center for Information Technology, NIH, Bethesda, MD
- Randall Pursley, Center for Information Technology, NIH, Bethesda, MD
- Hiroaki Suzuki, PhD, Hamamatsu Photonics, Hamamatsu, Japan
- Swee Lay Thein, MB, FRCP, FRCPath, DSc, Sickle Cell Branch, NHLBI, Bethesda, MD
Contact
For more information, email bruce.tromberg@nih.gov.