Regulation of Intracellular Iron Metabolism
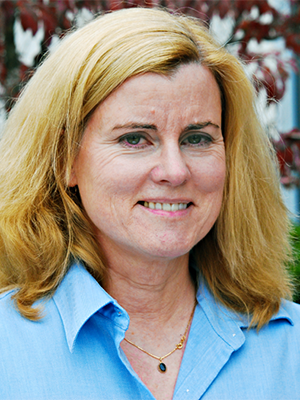
- Tracey A. Rouault, MD, Head, Section on Human Iron Metabolism
- Wing Hang Tong, PhD, Staff Scientist
- Deliang Zhang, PhD, Staff Scientist
- Manik C. Ghosh, PhD, Research Assistant
- Nunziata Maio, PhD, Visiting fellow
- Hayden Ollivierre-Wilson, Animal Care Technician
- Anamika Singh, MS, Technical Contract Worker
- Gregory Holmes-Hampton, PhD, Postdoctoral Trainee
- Shawn Huang, PhD, Postdoctoral Trainee
- Ki-Soon Kim, PhD, Postdoctoral Trainee
- Gennadiy Kovtunovych, PhD, Special Volunteer
Our goal is to understand how mammals regulate intracellular and systemic iron metabolism. Iron-regulatory proteins 1 and 2 (IRP1 and IRP2) regulate the expression of numerous proteins of iron metabolism. In iron-depleted cells, the proteins bind to RNA stem-loops in transcripts known as iron-responsive elements (IRE). IRP binding stabilizes the mRNA that encodes the transferrin receptor and represses the translation of transcripts that contain IREs near the 5′ end of the ferritin H and L chains. IRP1 is an iron-sulfur protein that functions as an aconitase in iron-replete cells. IRP2 is homologous to IRP1 but undergoes iron-dependent degradation in iron-replete cells. In mouse models, loss of IRP2 results in mild anemia, erythropoietic protoporphyria, and adult-onset neurodegeneration—all likely the result of functional iron deficiency. Biochemically and with expression arrays, we have studied the mechanisms that lead to anemia and neurodegeneration in IRP2−/− mice. We are using our mouse model of neurodegeneration to identify compounds that can prevent neurodegeneration; for example, we found that the antioxidant Tempol works by activating the latent IRE–binding activity of IRP1. We are evaluating the possibility that loss of IRP2 in humans causes mild refractory anemia and adult-onset neurodegeneration, which is characterized by limb weakness and might be diagnosed as amyotrophic lateral sclerosis. Given that mitochondrial energy production is required to maintain axonal integrity and that motor neurons have the longest and most vulnerable axons, we hypothesize that mitochondrial dysfunction resulting from iron deficiency causes axonal degeneration. We discovered that deficiency in IRP1 causes polycythemia and pulmonary hypertension owing to translational derepression of HIF2α through the IRE–IRP system. Our discovery introduces a new level of physiological regulation of erythropoiesis and provides a model for early pulmonary hypertension.
The molecular basis for regulation of intracellular iron metabolism in mammals
In previous years, our laboratory identified and characterized the cis and trans elements mediating iron-dependent alterations in the abundance of ferritin and the transferrin receptor. IREs are RNA stem-loops found in the 5′ end of ferritin mRNA and the 3′ end of transferrin receptor mRNA. We cloned, expressed, and characterized two essential iron-sensing proteins, IRP1 and IRP2. IRPs bind to IREs when iron levels are depleted, resulting in either inhibition of translation of ferritin mRNA and of other transcripts that contain an IRE in the 5′-untranslated regions (UTR) or stabilization of the transferrin receptor mRNA and possibly other transcripts that contain IREs in the 3′ UTR. The IRE–binding activity of IRP1 depends on the presence of an iron-sulfur cluster (see “Mammalian iron-sulfur cluster biogenesis” below). IRP2 also binds to IREs in iron-depleted cells but, unlike IRP1, is degraded in iron-replete cells, where it is selectively ubiquitinated and then degraded by the proteasome.
To approach questions about the physiology of iron metabolism, we generated loss-of-function mutations of IRP1 and IRP2 in mice through homologous recombination in embryonic cell lines. In the absence of provocative stimuli, we initially observed no abnormalities in iron metabolism associated with loss of IRP1 function. IRP2−/− mice develop a progressive neurologic syndrome characterized by gait abnormalities and axonal degeneration. Ferritin overexpression occurs in affected neurons and in protrusions of oligodendrocytes into the space created by axonal degeneration. IRP2−/− animals develop iron-insufficiency anemia and erythropoietic protoporphyria. In animals that lack IRP1, IRP2 compensates for loss of IRP1’s regulatory activity in most cell types, but we discovered several cell types and accompanying phenotypes in which IRP2 expression cannot be sufficiently increased to compensate. Animals that lack both IRP1 and IRP2 die as early embryos. The adult-onset neurodegeneration of adult IRP2−/− mice is exacerbated when one copy of IRP1 is also deleted. IRP2−/− mice offer a unique example of spontaneous adult-onset, slowly progressive neurodegeneration; analyses of gene expression and iron status at various stages of disease are ongoing. Dietary supplementation with the stable nitroxide Tempol prevents neurodegeneration; the treatment appears to work by recruiting IRE–binding activity of IRP1. We found that motor neurons were the most adversely affected neurons in IRP2−/− mice and that neuronal degeneration accounted for the gait abnormalities.
We discovered a form of the iron exporter ferroportin that lacks an IRE at its 5′ end and is important in permitting iron to cross the duodenal mucosa in iron-deficient animals and in preventing developing erythroid cells from consuming excessive amounts of iron in iron-deficient animals. In addition, we recently discovered that loss of IRP1 causes polycythemia and pulmonary hypertension through derepression of hypoxia-inducible factor 2-α (HIF-2α) translation in renal interstitial through the IRE–IRP system.
We also elucidated the pathophysiology of intravascular hemolysis and hyposplenism in animals that lack heme oxygenase 1. Their tissue macrophages die because they cannot metabolize heme after phagocytosis of red cells. To mitigate or reverse disease, we performed bone marrow transplants from wild-type animals to supply animals with functional macrophages; the bone marrow transplants were successful. We thus aim to correct the heme oxygenase defect in hematopoietic stem cells, using CRISPR technology, and to fully correct heme-oxygenase deficiency in the mice, experiments that would pave the way to treating heme oxygenase 1–deficient human patients, an underdiagnosed rare patient group.
Mammalian iron-sulfur cluster biogenesis
Our goals in studying mammalian iron-sulfur biogenesis are to understand how iron-sulfur prosthetic groups are assembled and delivered to target proteins in the various compartments of mammalian cells, including mitochondria, cytosol, and nucleus. In addition, we seek to understand the role of iron-sulfur cluster assembly in the regulation of mitochondrial iron homeostasis and the pathogenesis of diseases such as Friedreich’s ataxia and sideroblastic anemia, which are both characterized by incorrect regulation of mitochondrial iron homeostasis.
IRP1 is an iron-sulfur protein related to mitochondrial aconitase, which is a citric acid cycle enzyme that functions as a cytosolic aconitase in iron-replete cells. Regulation of RNA–binding activity of IRP1 involves a transition from a form of IRP1 in which a [4Fe-4S] cluster is bound to a form that loses both iron and aconitase activity. The [4Fe-4S]–containing protein does not bind to IREs. Controlled degradation of the iron-sulfur cluster and mutagenesis reveal that the physiologically relevant form of the RNA–binding protein in iron-depleted cells is an apoprotein. The status of the cluster appears to determine whether IRP1 binds to RNA. We identified numerous mammalian enzymes of iron-sulfur cluster assembly that are homologous to those encoded by the nifs, iscu, and nifu genes, which are implicated in bacterial iron-sulfur cluster assembly, and we observed that mutations in several iron-sulfur cluster biogenesis proteins cause disease. Loss of frataxin causes Friedreich's ataxia, which is characterized by progressive compromise of balance and cardiac function. In a cohort of patients of Swedish descent, we found that loss of ISCU causes skeletal myopathy. To explain the tissue specificity of ISCU myopathy, we studied myoblasts and other patient-derived tissue samples and cell lines. We discovered that many factors contribute to insufficiency of the iron-sulfur scaffold protein ISCU in skeletal muscle, including more pronounced abnormal splicing and unusual sensitivity of ISCU to degradation upon exposure to oxidative stress. Thus, oxidative stress may impair the ability of tissues to repair damaged iron-sulfur clusters by directly damaging a key component of the biogenesis machinery. A splicing abnormality of glutaredoxin 5 was found to be associated with sideroblastic anemia in one patient. In the affected tissues, mitochondrial iron overload is a feature common to all three diseases. In collaborative work, we discovered mutations of two new iron-sulfur cluster assembly proteins, NFU1 and BOLA3, which are required to provide iron-sulfur clusters to lipoate-dependent enzymes. We identified a tripeptide motif, LYR, in apoproteins that are recipients of nascent iron-sulfur clusters. The co-chaperone HSC2 binds to HSPA9, its partner HSP70–type chaperone, and that chaperone complex binds to ISCU that contains a nascent iron-sulfur cluster and two recipient proteins. We identified several direct iron-sulfur–recipient proteins in a yeast two-hybrid assay using HSC20 as bait. By studying one known iron-sulfur recipient, succinate dehydrogenase subunit B, we discovered that multiple LYR motifs of the SDHB primary sequence engage the iron-sulfur transfer apparatus by binding to the C-terminus of HSC20, facilitating delivery of the three iron-sulfur clusters of succinate dehydrogenase subunit B. We further discovered that the assembly factor SDHAF1 also engages the iron sulfur cluster transfer complex to facilitate transfer of iron sulfur clusters to SDHB. The discovery of the LYR motif will aid in identification of unknown iron-sulfur proteins, which are likely much more common in mammalian cells than has been previously appreciated.
Using expression arrays, we analyzed the mechanisms by which compromised mitochondrial iron-sulfur cluster biogenesis leads to mitochondrial iron overload. We postulate that regulation of mitochondrial iron homeostasis depends on intact synthesis of an iron-sulfur cluster–regulatory protein. Once this pathway is better understood, insights may lead to treatments for several rare diseases.
We are pursuing the use of anti-sense therapy as a treatment for ISCU myopathy, and we have been able to correct the causal splicing defect in patient myoblasts using stable anti-sense RNAs that were manufactured by high-quality techniques suitable for use in patients.
Publications
- Kovtunovych G, Ghosh MC, Ollivierre W, Weitzel RP, Eckhaus MA, Tisdale JF, Yachie A, Rouault TA. Wild-type macrophages reverse disease in heme oxygenase 1-deficient mice. Blood 2014; 124:1522-1530.
- Maio N, Singh A, Uhrigshardt H, Saxena N, Tong WH, Rouault TA. Iron-sulfur cluster biogenesis in mammalian cells: new insights into the molecular mechanisms of cluster delivery. Cell Metab 2014; 19:445-457.
- Rouault TA. Mammalian iron-sulphur proteins: novel insights into biogenesis and function. Nat Rev Mol Cell Biol 2015; 16:45-55.
- Ghosh MC, Zhang L, Rouault TA. Iron misregulation and neurodegenerative disease in mouse models that lack iron regulatory proteins. Neurobiol Dis 2015; 81:66-75.
- Rouault TA. Iron-sulfur proteins hiding in plain sight. Nat Chem Biol 2015; 11:442-445.
Collaborators
- Ronald Haller, MD, University of Texas Southwestern Medical Center, Dallas, TX
- W. Marston Linehan, MD, Urologic Oncology Branch, Center for Cancer Research, NCI, Bethesda, MD
- John F. Tisdale, MD, Molecular and Clinical Hematology Branch, NIDDK, Bethesda, MD
Contact
For more information, email trou@helix.nih.gov or visit https://science.nichd.nih.gov/confluence/display/rouault/Home.