Nervous System Development and Plasticity
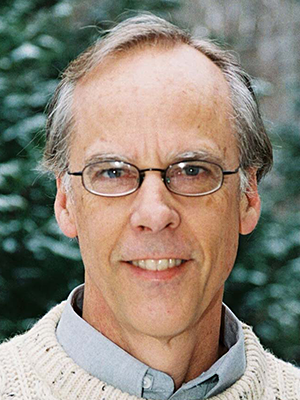
- R. Douglas Fields, PhD, Head, Section on Nervous System Development and Plasticity
- Philip Lee, PhD, Staff Scientist
- William Huffman, MA, Technician
- Dipankar Dutta, PhD, Visiting Fellow
- Jillian Belgrad, BA, Postbaccalaureate Fellow
- Nancy Orgega, BA, Undergraduate Scholarship Program Postbaccalaureate Research Fellow
Healthy brain and cognitive development in children is central to the mission of NICHD. Unlike the brains of most animals, the human brain continues to develop postnatally, through adolescence and into early adulthood. The prolonged postnatal period of brain development allows environmental experiences to influence brain structure and function, rather than having brain function specified entirely by genes. Activity-dependent plasticity also compensates for developmental defects and brain injury. Our research is concerned with understanding the molecular and cellular mechanisms by which functional activity in the brain regulates development of the nervous system during late stages of fetal development and early postnatal life. We are especially interested in novel mechanisms of activity-dependent nervous system plasticity that are particularly relevant to the period of childhood and of those that operate beyond the synapse and beyond the neuron doctrine. Our work has three main areas of emphasis: myelination and neuron-glia interactions, cellular mechanisms of learning, and gene regulation by neuronal firing.
Traditionally, the field of activity-dependent nervous system development has focused on synapses, and we continue to explore synaptic plasticity. However, our research is also advancing our understanding of how non-neuronal brain cells (glia) sense neural impulse activity and how activity-dependent regulation of glia contributes to development, plasticity, and the cellular mechanisms of learning. A major emphasis of our current research is to understand how myelin (white matter in the brain) is regulated by functional activity. By changing conduction velocity, activity-dependent myelination may be a non-synaptic form of plasticity, regulating nervous system function by optimizing the speed and synchrony of information transmission through neural networks. Our studies identified several cellular and molecular mechanisms for activity-dependent myelination, and the findings have important implications for normal brain development, learning and cognition, and psychiatric disorders. Our research showing that myelination of axons by glia (oligodendrocytes and Schwann cells) is regulated by impulse activity provides evidence for a new form of nervous system plasticity and learning that would be particularly important in child development, given that myelination proceeds throughout childhood and adolescence. The mechanisms we identified suggest that environmental experience may alter myelin formation in an activity-dependent manner, thereby improving function based on experience.
Learning is perhaps the most important function of childhood. Our research is delineating the molecular mechanisms that convert short-term memory into long-term memory. While we continue our long-standing research on synaptic plasticity, our laboratory is actively exploring new mechanisms of nervous system plasticity during learning that extend beyond the neuron doctrine, such as neurons firing antidromically (backwards) and the release of neurotransmitters along axons. We are investigating how gene expression necessary for long-term memory is controlled and how intrinsic activity in the brain (oscillations and neuronal firing) forms memories. Our recent research showed that neurons in the hippocampus fire antidromically during sharp-wave ripple complexes, which are most frequent during slow-wave sleep, and that the firing reduces the strength of all synapses on that neuron (action potential–induced long-term depression or AP-LTD).
Information in the nervous system is encoded in the temporal pattern of action potential firing. If functional experiences produce lasting effects on brain development and plasticity, specific genes must be regulated by specific patterns of impulse firing. We verified the hypothesis and are determining how various patterns of neural impulses regulate specific genes controlling development and plasticity of the nervous system and how impulse activity affects neurons and glia.
Regulation of myelination by neural impulse activity
Myelin, the multilayered membrane of insulation wrapped around nerve fibers (axons) by glial cells (oligodendrocytes), is essential for nervous system function, increasing conduction velocity at least 50-fold. Myelination is an essential part of brain development, but the processes controlling myelination of appropriate axons are not well understood. Myelination begins in late fetal life and continues throughout childhood and adolescence, but myelination of some brain regions is not complete until an individual's early twenties. Our research shows that neurotransmitters that are released along axons firing action potentials activate receptors on myelinating glia (Schwann cells in the peripheral nervous system and oligodendrocytes in the central nervous system) as well as on astrocytes and other cells, which in turn release growth factors, cytokines, and other molecules that regulate development of myelinating glia.
Induction of myelination by action potentials
In addition to establishing the effects of impulse activity on proliferation and development of myelinating glia, we determined that release of the neurotransmitter glutamate from vesicles along axons promotes the initial events in myelin induction, including stimulating the formation of cholesterol-rich signaling domains between oligodendrocytes and axons and increasing the local synthesis of myelin basic protein, the major protein in the myelin sheath, through Fyn kinase–dependent signaling. We showed that, through this axon-oligodendrocyte signaling mechanism, electrically active axons become preferentially myelinated by a factor of 8 to 1 over electrically inactive axons, thus regulating myelination of axons and neural circuit function according to functional experience. The findings are also relevant to such demyelinating disorders as multiple sclerosis and to remyelination after axon injury.
This research is expanding the biological significance of myelin. Myelin has been traditionally viewed in terms of conduction failure after damage, but we are exploring how myelin and changes in myelin affect spike time arrival, the frequency, phase, and amplitude coupling of oscillations in the brain, as well as the propagation of brain waves. Abnormalities in brain waves and synchrony are associated with many psychiatric and developmental conditions, including, among others, schizophrenia, epilepsy, dyslexia, and autism.
Modification of myelin structure and conduction velocity by astrocytes
Many neurological and psychological dysfunctions can develop when optimal synchrony of spike time arrival and appropriate conduction latencies that are required to sustain neural oscillations are disturbed. Given that, to achieve temporal summation at synapses, optimal neural circuit function and synaptic plasticity require the proper impulse transmission speed through all axons to induce spike timing–dependent plasticity, and to sustain oscillations at appropriate frequencies, mechanisms that determine and modify conduction time through axons could provide a non-synaptic mechanism of neural circuit plasticity. Conduction velocity in myelinated axons depends on the thickness of the myelin sheath and the morphology of electrogenic nodes of Ranvier along axons. Our research and that of others has shown that myelination of unmyelinated axons and the thickness of the myelin sheath can be increased in response to neural activity and environmental experience. Also, during growth of axons, the myelin sheath is thickened, but once formed, myelin structure was believed to be static. There was no known mechanism that could reduce the thickness of the mature myelin sheath, except in the context of pathology, but such a mechanism would be necessary to reduce conduction velocity to promote optimal spike time arrival from inputs that arrive at relay points in neural networks too soon.
Our research published this year shows that myelin thickness and nodal gap length are reversibly altered by astrocytes, the glial cells that contact nodes of Ranvier, and that this alters the speed of impulse transmission and neural network function. Myelin is attached to the axon by intercellular junctions adjacent to the node of Ranvier. We found that one of these cell adhesion molecules (neurofascin 155) has a binding site for the proteolytic enzyme thrombin, which is secreted by neurons and enters the brain from the vascular system. We found that thrombin-dependent cleavage of neurofascin 155 severs the tether between the axon and myelin, allowing it to detach and render the myelin sheath thinner. The process is inhibited by vesicular release of thrombin protease inhibitors from perinodal astrocytes. Previously, it was unknown how the myelin sheath could be thinned, and the functions of perinodal astrocytes were not well understood. The findings provide a new form of nervous system plasticity in which myelin structure and conduction velocity are adjusted by astrocytes. The thrombin-dependent cleavage of neurofascin 155 may also have relevance to myelin disruption and repair.

Click image to view.
Figure 1. Myelin plasticity to modulate impulse conduction velocity
The speed of neural impulse transmission is altered by astrocytes (aqua) at electrogenic nodes of Ranvier (grey), regulating detachment of the outer layers of the myelin sheath (purple) from the axon (tan) via thrombin-dependent cleavage of a cell adhesion molecule NF155. 3D reconstruction from electron microscopy.
Gulf War Illness
After decades of research, there is still no understanding of how a large group of Gulf War veterans became chronically ill with Gulf War Illness. It is believed that exposure to low levels of sarin nerve gas and combinations of organophosphate insecticides, which impair synaptic function, may be responsible. Our recent discovery that glutamatergic transmission between axons and oligodendrocytes triggers myelination led us to propose that impairments in myelination owing to disrupted neurotransmission from axons to oligodendrocytes may be an underlying cause of Gulf War Illness. Our research in progress is showing that proliferation and development of oligodendrocytes is affected in an animal model of Gulf War illness, and such perturbations could have long-lasting consequences in neural network functions involved in many of the symptoms associated with Gulf War Illness, including difficulties with working memory, mental focus, chronic pain, and others.
Synaptic plasticity
It is widely appreciated that there are two types of memory: short-term and long-term, and that sleep has a critical role in memory consolidation. Gene expression is necessary to convert short-term into long-term memory, but it is not known how signals reach the nucleus to initiate this process or which genes control strengthening and weakening of synapses in association with learning. Long-term potentiation (LTP) and long-term depression (LTD) are two widely studied forms of synaptic plasticity that can be recorded electrophysiologically in the hippocampus and are believed to represent a cellular basis for memory. We use electrophysiology, cDNA microarrays, and RNAseq to investigate the signaling pathways, genes, and proteins involved in LTP and LTD. The work is contributing to a better understanding of how regulatory networks are controlled by appropriate patterns of impulses, leading to different forms of synaptic plasticity, and is identifying new molecular mechanisms regulating synaptic strength.
Regulation of gene expression by action-potential firing patterns
To determine how gene expression in neurons and glia is regulated by impulse firing, we stimulate nerve cells to fire impulses in differing patterns by optogenetics and by delivering electrical stimulation through platinum electrodes in specially designed cell culture dishes. After stimulation, we measured mRNA and protein expression by gene microarrays, quantitative RT-PCR (reverse transcriptase–polymerase chain reaction), RNAseq, Western blot, and immunocytochemistry. The results confirm our hypothesis that precise patterns of impulse activity can increase or reduce expression of specific genes (in neurons and glia). Moreover, regulation of gene expression in neurons by specific temporal patterns of impulse activity is not a property of special genes; the neuronal transcriptome in general is highly regulated by the pattern of membrane depolarization, with hundreds of genes differentially regulated by the temporal code of neuronal firing. Our experiments are thus revealing the intracellular signaling and gene-regulatory networks that respond selectively to appropriate temporal patterns of action-potential firing. Temporal aspects of intracellular calcium signaling are particularly important in regulating gene expression according to neural-impulse firing patterns in normal and pathological conditions. Our findings provide a deeper understanding of how nervous system development and plasticity are regulated by information coded in the temporal pattern of impulse firing in the brain. The findings are also relevant to chronic pain as well as to the regulation of nervous system development and myelination by functional activity.
In collaboration with David Clark, we are investigating chromatin structure and remodeling in neurons and glia, and our work is revealing fundamental differences in chromatin structure between neurons and glia (astrocytes and oligodendrocytes).
Publications
- Dutta DJ, Woo DH, Lee PR, Pajevic S, Bukalo O, Huffman WC, Wake H, Basser PJ, Sheikhbahaei S, Lazarevic V, Smith JC, Fields RD. Regulation of myelin structure and conduction velocity by perinodal astrocytes. Proc Natl Acad Sci USA 2018;115(46):11832-11837.
- Lee PR, Cohen JE, Iacobas DA, Icobas S, Fields RD. Gene networks activated by specific patterns of action potentials in dorsal root ganglia neurons. Sci Rep 2017;7:43765.
- Fields RD, Dutta DJ, Belgrad J, Robnett M. Cholinergic signaling in myelination. Glia 2017;65:687-698.
- Belgrad J, Fields, RD. Epigenome interactions with patterned neuronal activity. Neuroscientist 2018;24:471-485.
- Martin JE, Nguyen TT, Grunseich C, Nofziger JH, Lee PR, Fields D, Fischbeck KH, Foran E. Decreased motor neuron support by SMA astrocytes due to diminished MCP1 secretion. J Neurosci 2017;37:5309-5318.
Collaborators
- Peter J. Basser, PhD, Section on Quantitative Imaging and Tissue Sciences, NICHD, Bethesda, MD
- David Clark, PhD, Section on Chromatin and Gene Expression, NICHD, Bethesda, MD
- Kenneth Fischbeck, MD, Neurogenetics Branch, NINDS, Bethesda, MD
- Vanja Lazarevic, PhD, Experimental Immunology Branch, Center for Cancer Research, NCI, Bethesda, MD
- James O'Callaghan, PhD, CDC Distinguished Consultant, NIOSH, and West Virginia University, Morgantown, WV
- Sinisa Pajevic, PhD, Division of Computational Bioscience, CIT, NIH, Bethesda, MD
- Shahriar Sheikhbahaei, PhD, Cellular and Systems Neurobiology Section, NINDS, Bethesda, MD
- Jeffrey C. Smith, PhD, Cellular and Systems Neurobiology Section, NINDS, Bethesda, MD
- Kimberly Sullivan, PhD, Boston University School of Public Health, Boston, MA
- Hiroaki Wake, PhD, National Institute for Basic Biology, Okazaki, Japan
Contact
For more information, email fieldsd@mail.nih.gov or visit http://nsdps.nichd.nih.gov.